Toward a consensus framework to evaluate air–sea CO2 equilibration for marine CO2 removal
Limnology and oceanography letters(2023)
摘要
Atmospheric carbon dioxide removal (CDR) is considered an essential component of climate change mitigation—as a supplement to emission reductions. Marine CDR methods have the potential to provide gigatonne-scale CDR but they differ from terrestrial methods in that they first reduce CO2 in seawater to ultimately increase the net flux of CO2 from the atmosphere to the ocean. For CDR to be realized, CO2 must be absorbed by seawater after the deployment of a marine CDR method. However, equilibration with atmospheric CO2 takes months to years and occurs over vast ocean regions, which raises concerns about whether the atmospheric CO2 influx can be robustly verified. Here, we suggest a “risk assessment for incomplete CO2 equilibration” as a pragmatic way to satisfy verification requirements. We illustrate the possible resources required for such a risk assessment, propose potential verification standards, and discuss research needs. Our Essay seeks to stimulate debate on how marine scientists can contribute to shaping and improving a potentially nascent marine CDR economy. Deliberately altering atmospheric CO2 to influence the Earth's climate has been debated for decades (Keith 2000), but was generally not considered a key strategy for climate mitigation. This viewpoint has changed since The Paris Agreement in 2015, and with the IPCC's 6th assessment report concluding in April 2022 that atmospheric CO2 removal (CDR) is now “unavoidable” to reach net zero emissions (IPCC 2022). The ocean has great potential to deliver CDR at scale but marine CDR (mCDR) methods have key constraints that differ critically from terrestrial CDR approaches. One of the most pronounced differences is that most mCDR methods create or enhance a seawater pCO2 (partial pressure of CO2) deficit when deployed in regions where the seawater pCO2 is equal to or below atmospheric pCO2. Or, they reduce a seawater pCO2 surplus in regions where seawater pCO2 is higher than atmospheric pCO2 (e.g., Eastern Boundary Upwelling Systems). However, in stark contrast to terrestrial CDR methods, mCDR methods cannot directly remove CO2 from the atmosphere. Atmospheric CO2 removal is only potentially occurring in a subsequent step, namely when surface seawater pCO2 equilibrates with atmospheric pCO2. Thus, atmospheric CO2 removal depends upon how completely atmospheric CO2 equilibrates with the CO2-deficient surface water following an mCDR deployment. Or, when the mCDR deployment is in a pCO2 surplus region, how much of the surplus CO2 would have outgassed into the atmosphere in the absence of the mCDR deployment. The necessary air–sea CO2 exchange depends on various factors such as the residence time of seawater at the surface, carbonate chemistry, and how rapidly atmospheric CO2 invades the surface ocean (Wanninkhof et al. 2009). Previous studies have argued that tracing air–sea CO2 flux is a key component of mCDR (Gnanadesikan and Marinov 2008; Williamson et al. 2012; Bach et al. 2021). These arguments are based on two reasons. First, there is a concern that the seawater CO2 deficit generated through an mCDR deployment will not be equilibrated for decades to centuries if mCDR is employed in some unfavorable oceanic regions, such as deep-water formation regions where water loses contact with the atmosphere for a long time (Jones et al. 2014; He and Tyka 2023; Fig. 1). Second, credible mCDR certificates will require rigorous monitoring, reporting and verification of atmospheric CO2 removal (Mace et al. 2021). Under these constraints, mCDR would in principle require quantification and verification of the air–sea CO2 flux that can be linked to individual mCDR operations. Tracing CO2 influx and linking it to an individual mCDR deployment via an observational approach would require tracking a patch of seawater that has an mCDR-derived CO2 deficit in a Lagrangian manner, possibly by using a chemical tracer such as sulfur hexafluoride (Bakker et al. 2005). To verify the CO2 influx using observations, we would need to measure (1) air–sea CO2 flux throughout the perturbed patch, (2) mixing and diffusion of dissolved inorganic carbon (DIC) from the surrounding waters into the perturbed seawater patch, (3) natural CO2 sources and sinks (or other mCDR deployments) within the patch that modify the CO2 deficit. Figure 1 shows that all three components would have to be monitored over an increasing ocean area as the mCDR-modified patch spreads and the CO2 deficit from the mCDR deployment becomes increasingly dilute. Thus, it would require measurements that can resolve fine-scale gradients, ideally in situ at the ocean basin scale. To give some sense of where we currently stand in terms of our ability to detect these basin-scale changes in carbon inventories: Because the ocean contains a large inventory of inorganic carbon (37,200 GtC; Keppler et al. 2020), a small change will be difficult to detect. For example, Wanninkhof et al. (2013) showed that they were unable to detect the ocean's decadal uptake of ~27 GtC of atmospheric CO2 using state-of-the-art methodology for directly measuring DIC since this represents such a small change in the total inventory of oceanic inorganic carbon. They were only able to detect the anthropogenic CO2 signal by discrete pCO2 measurements throughout the water column since the signal-to-noise of pCO2 is much larger due to the partitioning of inorganic carbon into carbonate, bicarbonate, and aqueous dissolved CO2. However, discrete pCO2 measurement, which is not to be confused with commonly made surface underway pCO2 measurement done with an equilibrator, is a difficult and time-consuming method that is rarely applied, and autonomous in situ pCO2 sensors are currently restricted to a depth of ~ 50 m and unsuitable for making measurements for ocean inventory of CO2. For much smaller-scale individual mCDR deployments, methods providing better precision would have to rely upon an armada of sensors that cover much of the surface ocean with sufficient spatial and temporal resolution. A potentially high number of these sensors would be needed per km2 to account for the relevant submesoscale fluid variability (McWilliams 2016) and the surface ocean cover ~ 360 million km2. Based on the above reasons, we assume that quantifying/verifying CO2 flux via direct observations and linking it to individual mCDR operations is not possible in the near- and medium-term future. Thus, insisting on real-world quantification of these fluxes to satisfy verification needs would essentially cut off the access of mCDR to financing, endanger the development of mCDR, and ultimately limit its potential to contribute to the global CDR portfolio. Considering the potential of mCDR deployments to sequester atmospheric CO2, losing it as part of the CDR portfolio would be a blow to the goal to achieve net zero emissions. Therefore, mCDR deployments must become able to satisfy the need for robust verification of air–sea CO2 flux but without the requirement for direct measurement of CDR-induced CO2 fluxes. Here, we suggest “a risk assessment for incomplete near-term equilibration” as a pragmatic way forward. In this framework, mCDR deployments would not need to trace the influx of CO2 explicitly. Instead, one would have to robustly evaluate the risk for incomplete equilibration before the deployment (using modeling as will be detailed in the following paragraph). “Complete” or “incomplete” could be defined in different ways. The simplest is the assignment of a categorical value that needs to be achieved after a set period, for example, 95% atmospheric equilibration of the initial seawater CO2 deficit within 10 yr of the mCDR deployment (note that 95% in 10 yr is perhaps a reasonable but ultimately arbitrary definition used here to explore this scenario). Setting a target of 10 yr would be consistent with the need to realize CDR within decades (IPCC 2022). This approach would also be aligned with common (although perhaps undesirable) practices from terrestrial afforestation where CO2 removal is accounted for when the seedling is planted even though CDR is anticipated to take place in the years to decades after, when the tree grows (Lefebvre et al. 2021). The 95% “completeness” can be considered as “almost all” with an arguably acceptable further 5% influx sometime after 10 yr. In this case, an mCDR credit (worth 100% of the amount of CO2 removed from seawater) could be claimed if equilibration goes beyond 95% but be withheld if not meeting this criterion within 10 yr (Fig. 2A). A more sophisticated approach is determining the explicit equilibration in the 10 yr since the mCDR deployment. For example, if a generated CO2 deficit is 80% re-equilibrated after 10 yr, then an mCDR credit would be worth 80% of the initially generated seawater CO2 deficit (Fig. 2B). A third way is refraining from any temporal threshold but instead incrementally building up the value of the mCDR credit depending on the degree of equilibration after the deployment. Here, the value of an mCDR credit would be influenced by the dynamics of the equilibration until theoretically 100% is attained at some time in the future (Fig. 2C). The practical limitations of real-world CO2 influx quantifications highlighted above will mean that numerical models will become the dominant approach for the proposed risk assessment (Watson et al. 2008). This requires a multiscale model capacity that can be used to determine small-scale processes and global overturning water mass movements. All of these models require fit-for-purpose air–sea CO2 flux parameterization for open ocean and coastal areas (Ho et al. 2006; Dobashi and Ho 2023). The coordinated evaluation of multiple model ensembles would be essential to provide insights into uncertainties and model spread in the CO2 equilibration timescales (Keller et al. 2018b). There will also be the need for field research (e.g., with deliberate tracer release studies) to provide new data that enables innovation and improvement of the applied modeling framework. Furthermore, field data and their assimilation in models can correct model biases and generate explicit uncertainty estimates (Lahoz and Schneider 2014; Carroll et al. 2020). It will be important to commence such a risk assessment as soon as possible with available modeling frameworks, but constantly improve our modeling tools through technological developments and close collaboration of model developers with observational teams. Indeed, this approach has been successful before as evidenced in “the quiet revolution of numerical weather predictions” (Bauer et al. 2015). Figure 1 shows numerical simulations for four ocean regions where the risk of incomplete air–sea CO2 flux was tested. For each region we simulated a deficit in seawater DIC of 0.25 Tmol at the start, spread over an ~ 5 × 5° area in the upper 10 m of the water column. A removal of DIC while leaving alkalinity unchanged is equivalent to seawater CO2 removal which is why we will refer to a simulated “CO2 deficit” in the following. The seawater CO2 deficit relates to the atmosphere such that the ocean has the capacity to take up atmospheric CO2 until being fully equilibrated and uptake stops. A CO2 deficit in the surface ocean could be achieved by any mCDR method that reduces seawater pCO2. This includes but is not limited to Ocean Alkalinity Enhancement where CO2 is “removed” by conversion to bicarbonate or Iron Fertilization and Seaweed Sinking where CO2 is “removed” by photosynthetic CO2-fixation with subsequent export into the deep ocean. The simulation was performed using the ECCO LLC270 0.3 × 0.3° model (Forget et al. 2015; He and Tyka 2023) using a simple five-tracer biogeochemical model (Dutkiewicz et al. 2005). The simulations were compared to a control run where no CO2 deficit was simulated. The difference between the control and the CO2 deficit runs shows the spreading, dilution, and continuous re-equilibration over 25 yr. Atmospheric CO2 was held constant at 415 ppm. Thus, we are not simulating a fully coupled Earth system and our model does not account for feedbacks from other carbon pools which can reduce the air–sea CO2 flux (Keller et al. 2018a). Indeed, studies with fully coupled Earth system models have revealed that the transfer of CO2 from the atmosphere into the ocean via mCDR reduces natural marine CO2 uptake, or can even turn other carbon pools (e.g., terrestrial pools) into CO2 sources (Oschlies 2009; Oschlies et al. 2010). This means that the approach chosen here is limited in that the amount of CO2 that can be taken up by the oceans after an mCDR operation is not identical to the CO2 deficit it created (the uptake is likely lower and equilibration timescales shorter than our results in Fig. 1 suggest). We fully acknowledge these limitations and emphasize the need for modeling that goes much further beyond our illustrative examples. For example, by including the above-mentioned interactive feedbacks between major carbon pools (Oschlies 2009) or improved representation of locations through regional modeling (Wang et al. 2023). The results of our simulations suggest that there are pronounced differences in the risk for incomplete CO2 equilibration (Fig. 1). For example, CO2 equilibration increases slowly after Year 1 and is only 48% complete at Year 25 when a CO2 deficit is simulated south of Iceland (Fig. 1A). Here, formation of North Atlantic Deep Water exports waters with the CO2 deficit to depth before most of the CO2 equilibration can happen, suggesting this area is poorly suited for mCDR. In contrast, a CO2 deficit simulated near Fortaleza, Brazil, becomes 95% equilibrated with atmospheric CO2 within 3 yr, an ideal case for mCDR deployments with respect to air–sea CO2 influx (Fig. 1B). Hawaii and Eastern Australia demonstrate intermediate behaviors with the former achieving 95% equilibration but only after 12 yr and the latter stalling at 92% equilibration (Fig. 1C,D). The use of models enables assessment of the risk for incomplete atmospheric CO2 influx (Fig. 1). Any of the three approaches proposed above and illustrated in Fig. 2 could be used (also other and potentially better approaches, not conceived here, could be employed). However, the value of the information obtained is constrained by the model skill and must be regarded with care. If there is limited trust in the capability of the model it may be safer to evaluate the risk based on the simpler “categorical” approach (Fig. 2A). Here, the idea is to implement a rather strict yes-or-no threshold so that only regions that achieve very high levels of equilibration pass the test, whereas the others are labeled “not suitable” with regards to air–sea CO2 equilibration. That way, potential mCDR deployment regions where equilibration is less certain are more likely to be filtered out (but there is also a higher risk of unnecessarily filtering out potentially useful regions). The incremental approaches as in Fig. 2B,C, unequivocally, put more trust in the model result. Here, the value of an mCDR credit would scale directly with the model output. This is, in theory, the most desirable information, but there it requires a high model skill because the model outcome gets enormous weight in the value assessment of an mCDR credit. Insufficient regulation of the proposed “risk assessment for incomplete CO2 equilibration” could lead to poor accounting practices and a general distrust of mCDR methods. In US forest carbon accounting, for example, CDR project developers could choose between the available carbon accounting protocols depending on which is most beneficial to the value of their carbon credits (Gifford 2020). This practice opens the door to misuse and should not be adopted for any sort of measurement, reporting, and verification in mCDR (including the verification of air–sea CO2 flux as the focus of this manuscript). One potential solution to prevent misuse would be a “separation of power,” where the mCDR deployment (analogues to the executive power) and the CO2 influx assessment (jurisdiction) would be fully independent. The accounting standards and necessary knowledge base for the influx assessment could be synthesized by an independent organization, which is funded internationally and ideally supported by the marine science community. As in the IPCC process, a team of scientists, with complementary skillsets, could cyclically check the validity of resources used for the proposed risk assessment, estimate uncertainties, and monitor its improvement. What could the most useful resources for the risk assessment look like? We envision a gridded dataset that provides the risk assessment and modeled uncertainty according to agreed-upon evaluation criteria (e.g., in Fig. 2) for any location in the oceans at different seasons. In essence, this would become an interactive ‘lookup’ map, where each grid field is linked to re-equilibration dynamics and timescales as in Fig. 2. This resource could then be used by mCDR project developers to identify where in the oceans and in which season their deployment is most suited with respect to the risk of insufficient air–sea CO2 equilibration. It could be applied to all mCDR methods that generate a seawater CO2 deficit (or reduce a seawater CO2 surplus) in the surface ocean although not to those that alter ocean physics in a major way (e.g., artificial upwelling). The gridded dataset would be maintained and further developed by the independent organization mentioned above to account for changes in this risk due to, for example, interannual variability, climate change, or improved parameterisations of air–sea CO2 exchange. Formalizing this dataset into international carbon accounting protocols would provide transparency for the public, security for the mCDR project developer, and reduce the risk for the operation of mCDR markets. Obviously, a gridded dataset, developed and reviewed with the necessary scrutiny, is not readily available. It would also require substantial, sustained, and independent funding support to provide the best possible data. However, we believe that aiming for such a resource would be fruitful for our understanding of the marine carbon cycle and an important contribution by the marine biogeochemistry community to help enable “unavoidable” gigatonne-scale atmospheric CO2 removal. Indeed, there is currently hardly any framework for mCDR certification (Arcusa and Sprenkle-Hyppolite 2022). This vacuum provides a window of opportunity for the marine science community to help shape the best-possible certification standards for a potentially nascent mCDR economy. Data S1. Supplementary methods: description of the modelling Please note: The publisher is not responsible for the content or functionality of any supporting information supplied by the authors. Any queries (other than missing content) should be directed to the corresponding author for the article. LTB acknowledges funding from the Australian Research Council through a Future Fellowship award (FT200100846). DTH acknowledges the Chaire de Recherche ENS-Chanel for his sabbatical stay at École Normale Supérieure in Paris. PWB was funded by the Australian Research Council with a Laureate award (FL160100131). The authors acknowledge formal reviews from Matt Long, Dustin Carroll, Andreas Oschlies, three other anonymous reviewers, the associate editor, and the editor.
更多查看译文
关键词
air–sea,marine,air–sea,equilibration
AI 理解论文
溯源树
样例
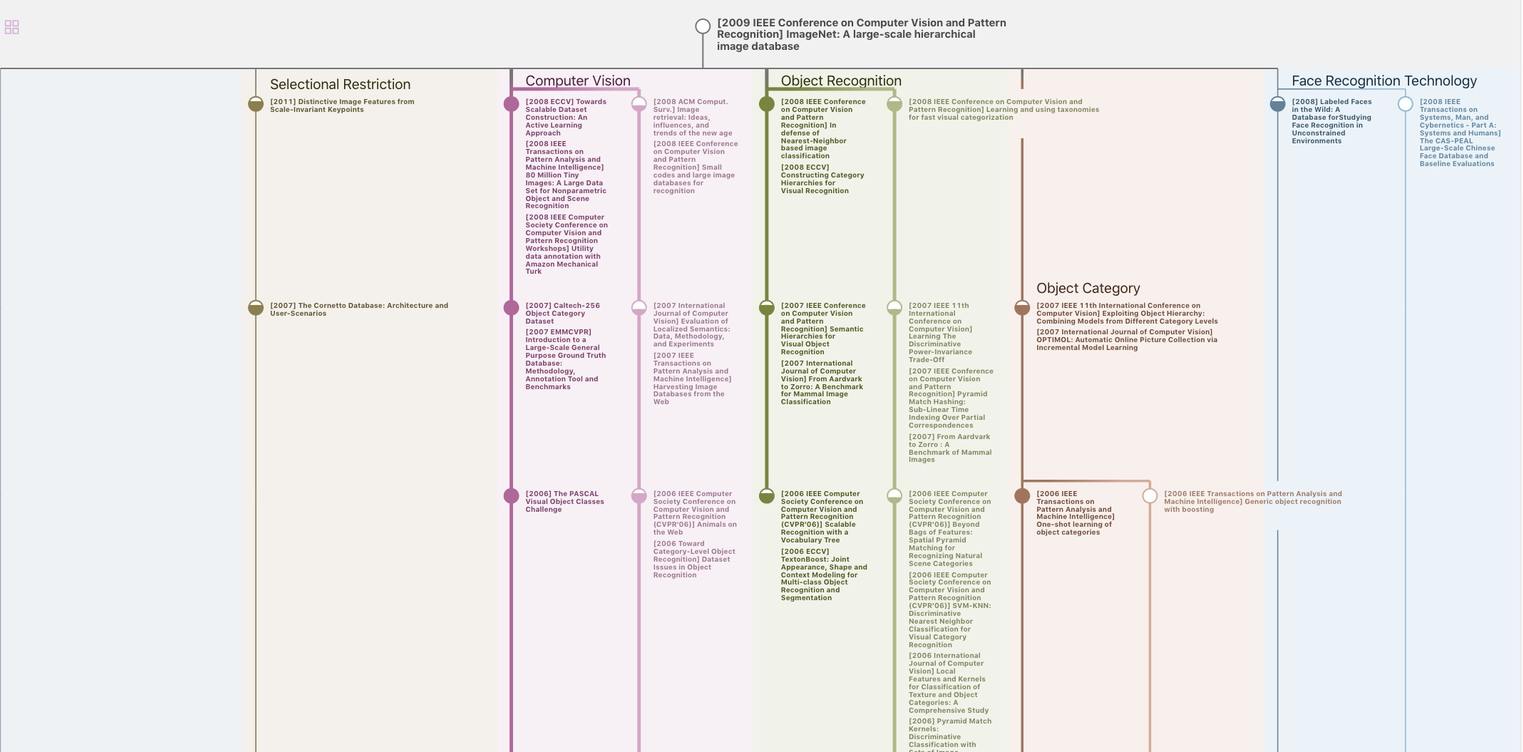
生成溯源树,研究论文发展脉络
Chat Paper
正在生成论文摘要