Termination of non‐coding transcription in yeast relies on both an RNA Pol II CTD interaction domain and a CTD‐mimicking region in Sen1
The EMBO Journal(2020)
摘要
Article28 February 2020Open Access Transparent process Termination of non-coding transcription in yeast relies on both an RNA Pol II CTD interaction domain and a CTD-mimicking region in Sen1 Zhong Han Zhong Han Université de Paris, CNRS, Institut Jacques Monod, Paris, France Université Paris-Saclay, Yvette, France Search for more papers by this author Olga Jasnovidova Olga Jasnovidova CEITEC-Central European Institute of Technology, Masaryk University, Brno, Czechia Search for more papers by this author Nouhou Haidara Nouhou Haidara Université de Paris, CNRS, Institut Jacques Monod, Paris, France Université Paris-Saclay, Yvette, France Search for more papers by this author Agnieszka Tudek Agnieszka Tudek Université de Paris, CNRS, Institut Jacques Monod, Paris, France Search for more papers by this author Karel Kubicek Karel Kubicek CEITEC-Central European Institute of Technology, Masaryk University, Brno, Czechia Search for more papers by this author Domenico Libri Domenico Libri orcid.org/0000-0001-6728-0594 Université de Paris, CNRS, Institut Jacques Monod, Paris, France Search for more papers by this author Richard Stefl Richard Stefl orcid.org/0000-0003-0558-5952 CEITEC-Central European Institute of Technology, Masaryk University, Brno, Czechia Search for more papers by this author Odil Porrua Corresponding Author Odil Porrua [email protected] orcid.org/0000-0002-3639-4027 Université de Paris, CNRS, Institut Jacques Monod, Paris, France Search for more papers by this author Zhong Han Zhong Han Université de Paris, CNRS, Institut Jacques Monod, Paris, France Université Paris-Saclay, Yvette, France Search for more papers by this author Olga Jasnovidova Olga Jasnovidova CEITEC-Central European Institute of Technology, Masaryk University, Brno, Czechia Search for more papers by this author Nouhou Haidara Nouhou Haidara Université de Paris, CNRS, Institut Jacques Monod, Paris, France Université Paris-Saclay, Yvette, France Search for more papers by this author Agnieszka Tudek Agnieszka Tudek Université de Paris, CNRS, Institut Jacques Monod, Paris, France Search for more papers by this author Karel Kubicek Karel Kubicek CEITEC-Central European Institute of Technology, Masaryk University, Brno, Czechia Search for more papers by this author Domenico Libri Domenico Libri orcid.org/0000-0001-6728-0594 Université de Paris, CNRS, Institut Jacques Monod, Paris, France Search for more papers by this author Richard Stefl Richard Stefl orcid.org/0000-0003-0558-5952 CEITEC-Central European Institute of Technology, Masaryk University, Brno, Czechia Search for more papers by this author Odil Porrua Corresponding Author Odil Porrua [email protected] orcid.org/0000-0002-3639-4027 Université de Paris, CNRS, Institut Jacques Monod, Paris, France Search for more papers by this author Author Information Zhong Han1,2,4, Olga Jasnovidova3,5, Nouhou Haidara1,2, Agnieszka Tudek1,6, Karel Kubicek3, Domenico Libri1, Richard Stefl3 and Odil Porrua *,1 1Université de Paris, CNRS, Institut Jacques Monod, Paris, France 2Université Paris-Saclay, Yvette, France 3CEITEC-Central European Institute of Technology, Masaryk University, Brno, Czechia 4Present address: Francis Crick Institut, London, UK 5Present address: Max Planck Institute for Molecular Genetics, Berlin, Germany 6Present address: Department of Biophysics, Institute of Biochemistry and Biophysics, Polish Academy of Sciences, Warsaw, Poland *Corresponding author. Tel: +33 (0) 157278035; E-mail: [email protected] The EMBO Journal (2020)39:e101548https://doi.org/10.15252/embj.2019101548 Correction added on 1 April 2020, after first online publication: This affiliation was corrected because the name of the university changed. PDFDownload PDF of article text and main figures. Peer ReviewDownload a summary of the editorial decision process including editorial decision letters, reviewer comments and author responses to feedback. ToolsAdd to favoritesDownload CitationsTrack CitationsPermissions ShareFacebookTwitterLinked InMendeleyWechatReddit Figures & Info Abstract Pervasive transcription is a widespread phenomenon leading to the production of a plethora of non-coding RNAs (ncRNAs) without apparent function. Pervasive transcription poses a threat to proper gene expression that needs to be controlled. In yeast, the highly conserved helicase Sen1 restricts pervasive transcription by inducing termination of non-coding transcription. However, the mechanisms underlying the specific function of Sen1 at ncRNAs are poorly understood. Here, we identify a motif in an intrinsically disordered region of Sen1 that mimics the phosphorylated carboxy-terminal domain (CTD) of RNA polymerase II, and structurally characterize its recognition by the CTD-interacting domain of Nrd1, an RNA-binding protein that binds specific sequences in ncRNAs. In addition, we show that Sen1-dependent termination strictly requires CTD recognition by the N-terminal domain of Sen1. We provide evidence that the Sen1-CTD interaction does not promote initial Sen1 recruitment, but rather enhances Sen1 capacity to induce the release of paused RNAPII from the DNA. Our results shed light on the network of protein–protein interactions that control termination of non-coding transcription by Sen1. Synopsis Pervasive transcription producing non-coding RNAs without function poses a threat to proper gene expression. Here, molecular and functional characterization reveals protein-protein interactions that are critical for timely termination of non-coding transcription by the conserved helicase Sen1 in yeast. Sen1 possesses a motif that mimics the C-terminal domain (CTD) of RNA polymerase II. Nrd1 interaction with the Sen1 CTD-mimicking region enhances Sen1 recruitment to noncoding RNAs. Sen1 recruitment to its RNA targets can also be Nrd1-independent. Sen1 interaction with the RNA polymerase II CTD is critical for transcription termination at non-coding genes. Introduction The concept of pervasive transcription emerged over a decade ago upon the discovery that a large fraction of both the prokaryotic and the eukaryotic transcriptomes is composed of non-coding RNAs (ncRNAs) without any obvious function. Pervasive transcription is potentially harmful for cell homeostasis since it can interfere with normal transcription of canonical genes and provoke the accumulation of toxic RNAs. Therefore, all organisms studied to date have evolved different mechanisms to circumvent the negative consequences of pervasive transcription. These mechanisms often rely on transcription termination and RNA degradation (for review, see Jensen et al, 2013). In Saccharomyces cerevisiae, there are two major pathways for termination of RNA polymerase II (RNAPII) transcription. A pathway that depends on a macromolecular complex including the cleavage and polyadenylation factor (CPF) is essentially responsible for transcription termination at protein-coding genes, whereas the Nrd1-Nab3-Sen1 (NNS) complex targets a large fraction of the non-coding RNAs (ncRNAs) produced in the cell. Specifically, the NNS complex terminates transcription of most snoRNAs and a class of ncRNAs dubbed CUTs, for cryptic unstable transcripts, that constitutes the major product of pervasive transcription (for review, see Porrua & Libri, 2015). While snoRNAs are important for the correct modification of rRNA, CUTs are generally considered as non-functional molecules (Wyers et al, 2005; Arigo et al, 2006; Thiebaut et al, 2006; Schulz et al, 2013). Each pathway is associated with distinct nuclease and polyA-polymerase activities that determine the stability and functionality of the RNAs they take care of. Precursors of mRNAs are cleaved at their 3′ ends at the so-called polyA site and polyadenylated by Pap1, which stimulates subsequent export to the cytoplasm and translation (for review, see Porrua & Libri, 2015). In contrast, ncRNAs terminated by the NNS-dependent pathway are polyadenylated by Trf4, a component of the TRAMP complex. These RNAs are then targeted by the nuclear form of the exosome bearing the Rrp6 exonuclease, which catalyses either 3′ end maturation, in the case of snoRNAs, or complete degradation, in the case of CUTs (LaCava et al, 2005; Vanacova et al, 2005; Wyers et al, 2005). Given the very divergent fate of the RNAs terminated by each pathway, several mechanisms have evolved to ensure the specific action of the different protein complexes on the right targets. These mechanisms involve both protein–protein and nucleic acid–protein interactions. Some of the protein interactions that are crucial for coordinated and efficient transcription-related processes as termination and 3′ end processing are mediated by the C-terminal domain (CTD) of the largest subunit of RNAPII. The CTD is a large and flexible domain composed of 26 repeats of the heptapeptide YSPTSPS that undergoes dynamic phosphorylation throughout the transcription cycle (for review, see Harlen & Churchman, 2017a). The CPF complex interacts preferentially with the S2P-form of the CTD, which is more prominent during middle-late elongation, via the CTD interaction domain (CID) of its associated factor Pcf11 (Lunde et al, 2010). In addition, the recognition of several AU-rich sequences, among which the polyA site, by other subunits, mediates the specific recruitment of the CPF complex to mRNAs (Xiang et al, 2014). Similarly, the CID of Nrd1 within the NNS complex interacts with the CTD phosphorylated at S5, which is a mark of early elongation (Vasiljeva et al, 2008; Kubicek et al, 2012), and both Nrd1 and Nab3 recognize specific sequence motifs that are enriched at the target ncRNAs (Hobor et al, 2011; Lunde et al, 2011; Wlotzka et al, 2011; Porrua et al, 2012; Schulz et al, 2013). Both the interaction with the CTD and with the nascent RNA contribute to the early recruitment of the Nrd1-Nab3 heterodimer and the efficiency of transcription termination (Gudipati et al, 2008; Vasiljeva et al, 2008; Tudek et al, 2014). The current model posits that Nrd1-Nab3 would recruit the helicase Sen1 that in turn promotes the final step in transcription termination (i.e. the release of RNAPII and the nascent RNA from the DNA, Porrua & Libri, 2013). Subsequently, a complex network of possibly redundant protein–protein interactions involving Nrd1, Nab3 and different components of the TRAMP and exosome complexes promotes efficient degradation of the released ncRNA (Tudek et al, 2014; Fasken et al, 2015; Kim et al, 2016). As mentioned above, Sen1 is responsible for dissociation of the elongation complex. Sen1 is a highly conserved RNA and DNA helicase belonging to the superfamily 1 of helicases (Martin-Tumasz & Brow, 2015; Han et al, 2017). Transcription termination by Sen1 involves its translocation along the nascent RNA towards RNAPII and possibly subsequent contacts between specific regions of Sen1 helicase domain and the polymerase (Porrua & Libri, 2013; Han et al, 2017; Leonait≐ et al, 2017). Neither in vitro nor in vivo Sen1 exhibits any sequence-specific RNA-binding capability (Creamer et al, 2011; Porrua & Libri, 2013); implying that Sen1 activity should be regulated in order to ensure its specific action on ncRNAs. Among the mechanisms that might contribute to keep Sen1 under control, we can cite: (i) the relatively low levels of Sen1 protein (63–498 molecules/cell depending on the study Ghaemmaghami et al, 2003; Newman et al, 2006; Kulak et al, 2014; Chong et al, 2015); (ii) its low processivity as a translocase, which makes termination highly dependent on Sen1 efficient recruitment to the elongation complex and RNAPII pausing (Han et al, 2017) and (iii) the interaction of Sen1 with the ncRNA targeting proteins Nrd1-Nab3, as evoked above. Although it has been proposed that Nrd1 and Nab3 function as adaptors that provide the necessary specificity to Sen1, the precise regions involved in the interaction between these factors and whether these interactions are actually sufficient for timely Sen1 recruitment remains unclear. In this study, we identify and characterize the key interactions involved in Sen1 function. Sen1 is composed of a central helicase domain [amino acids (aa) 1,095–1,876] that is sufficient for transcription termination in vitro (Han et al, 2017; Leonait≐ et al, 2017), together with a large N-terminal domain (aa 1–975) and a C-terminal intrinsically disordered region (1,930–2,231). Here, we show that the C-terminal end of Sen1 contains a short motif that mimics the phosphorylated CTD and is recognized by Nrd1 CID. We prove that this motif is the main determinant of the interaction between Sen1 and Nrd1-Nab3 heterodimer and provide the structural details of this interaction. Strikingly, we find that the CTD mimic in Sen1 is not a strict requirement for Sen1 function, although it contributes to Sen1 recruitment and to fully efficient termination at some targets. Instead, we show that the N-terminal domain of Sen1 promotes its interaction with the CTD of RNAPII and that this interaction is a global requirement for non-coding transcription termination under normal conditions. However, decreasing the transcription elongation rate renders the N-terminal domain less necessary for termination, which supports the notion that the Sen1-CTD interaction favours Sen1 action in the context of a kinetic competition between termination and elongation. We also find that the N-terminal and the C-terminal domains of Sen1 can interact with each other in vitro, which might modulate the interaction of Sen1 with RNAPII CTD and/or Nrd1. Our findings allow us to propose a detailed molecular model on how protein interactions can control the specific function of the transcription termination factor Sen1 on ncRNAs. Results Sen1 possesses a CTD mimic that is recognized by the CID domain of Nrd1 In a previous report (Tudek et al, 2014), we showed that Nrd1 CID domain can recognize a short sequence in Trf4 that mimic the S5P-CTD of RNAPII and that we dubbed NIM for Nrd1-Interaction Motif. A subsequent report described a second NIM in Mpp6, an exosome cofactor (Kim et al, 2016). During the course of our previous work, we discovered that the CID is also required for the interaction between Nrd1 and Sen1 (Fig 1A), an observation that was reported in an independent study (Heo et al, 2013). This prompted us to search for a putative NIM in Sen1 protein. The S5P-CTD and Trf4 NIM share three important features: (i) they contain one or several negatively charged aa at the N-terminal portion that interact with a positively charged surface of the CID; (ii) they contain a Y residue followed by several aa at the C-terminal part that adopt a β-turn conformation and interact with a hydrophobic pocket of the CID; and (iii) they are placed in protein regions that are predicted to be intrinsically disordered and therefore are fully accessible for the interaction with the CID. We identified a sequence in Sen1 C-terminal domain that fulfils the three characteristics and closely resembles the NIM in Trf4 (Fig 1B). Therefore, we tested the role of this motif by comparing the ability of wild type (wt) or ∆NIM versions of Sen1 to interact with Nrd1 by in vivo coimmunoprecipitation experiments using Nrd1-TAP as the bait (Fig 1C). Importantly, deletion of the putative NIM did not significantly alter the levels of Sen1 protein but dramatically reduced its interaction with Nrd1. Similar experiments using Sen1 as the bait confirmed these results and showed that deletion of the NIM also strongly affects the association of Sen1 with Nab3 (Fig 1D). These results indicate that Sen1 NIM is the main determinant of the interaction of Sen1 with the Nrd1-Nab3 heterodimer. They also strongly suggest that Nab3 interacts with Sen1 via Nrd1. Figure 1. Identification of a Nrd1-Interaction Motif (NIM) in Sen1 that is critical for the integrity of the NNS complex A. Deletion of the CID domain dramatically reduces the interaction of Nrd1 with Sen1. Coimmunoprecipitation (CoIP) experiments using TAP-tagged Nrd1 (either wt or ∆CID) as the bait. Representative gel of one out of two independent experiments. B. Scheme of Sen1 protein. Globular domains are denoted by solid bars, whereas intrinsically disordered regions are shown by a line. The disorder prediction was obtained using IUPred (Dosztányi et al, 2005). The sequence of the RNAPII S5P-CTD and Trf4 and Sen1 NIMs is shown on the top. Structural elements that are important for the interaction with Nrd1 CID are indicated. Conserved positions are underlined. C. Deletion of the NIM decreases substantially the association of Nrd1 with Sen1. CoIP experiments using Nrd1-TAP as the bait in a SEN1 or sen1∆NIM background. Representative gel of one out of two independent experiments. D. CoIP experiments using HA-tagged Sen1, either wt or ∆NIM, as the bait. Representative gel of one out of two independent experiments. Protein extracts were treated with RNaseA prior to immunoprecipitation. In these experiments, Sen1 could not be detected in the input extracts. Data information: Antibodies used for protein detection are listed in Appendix Table S3. Download figure Download PowerPoint The NIM is one of the very few sequence regions of the C-terminal domain of Sen1 that are conserved in the closest S. cerevisiae relatives, suggesting that this mode of interaction between Sen1 and Nrd1 is conserved in these yeast species (Fig EV1). Conversely, in agreement with previous data showing that Nrd1 and Sen1 orthologues do not interact with each other in Schizosaccharomyces pombe (Lemay et al, 2016; Wittmann et al, 2017) and with the fact that no Nrd1 homologue could be identified in association with human Sen1(Yüce & West, 2013), we did not detect any putative NIM in Sen1 orthologues from these organisms. Click here to expand this figure. Figure EV1. The Sen1 NIM is conserved in close yeast speciesSaccharomyces cerevisiae Sen1 protein sequence was submitted to blastp excluding the genus Saccharomyces from the search. The ten most conserved protein sequences together with Sen1 orthologues from Homo sapiens (SETX) and Schizosaccharomyces pombe were aligned to S. cerevisiae Sen1 using clustal omega. Visualization of the alignment and calculation of the consensus sequences ware performed with Jalview (Waterhouse et al, 2009). Amino acids are coloured according to clustal colour scheme. The protein identifiers for the proteins used in the alignment are the following: Q7Z333 (H. sapiens), Q92355 (S. pombe), XP_001644478.1 (Vanderwaltozyma polyspora), SMN21961.1 (Kazachstania saulgeensis), SCV99407.1 (Lachancea fermentati), CCK69072.1 (Kazachstania naganishii), XP_004181671.1 (Tetrapisispora blattae), XP_003955130.1 (Kazachstania Africana), XP_003672383.1 (Naumovozyma dairenensis), Q00416 (S. cerevisiae), XP_003680903.1 (Torulaspora delbrueckii), CDF91445.1 (Zygosaccharomyces bailii) and GAV52597.1 (Zygosaccharomyces rouxii). The NIM is indicated by a red box. Download figure Download PowerPoint Structural analyses of the Nrd1 CID–Sen1 NIM interaction To compare the interaction of the newly identified Sen1 NIM (fragment harbouring aa 2,052–2,063) and the previously identified Trf4 NIM with Nrd1 CID, we performed a quantitative solution-binding assay using fluorescence anisotropy (FA) with purified recombinant Nrd1 CID and synthetic NIM peptides. We found that Nrd1 CID binds Sen1 NIM with a KD of 1.2 ± 0.02 μM, which is comparable to the dissociation constant of the Trf4 NIM–Nrd1 CID complex (KD 0.9 ± 0.02 μM), and roughly 100-fold smaller than the KD of the Nrd1 CID for the S5P-CTD (Tudek et al, 2014). In addition, we observed that the binding is similarly affected by previously described mutations in Nrd1 CID (L20D, K21D, S25D, R28D, M126A, I130K, R133A, see Appendix Table S1). The similar binding strength was also confirmed by [1H,15N] heteronuclear single quantum coherence (HSQC) titration experiment of Nrd1 CID. In this experiment, the protein amide resonances of Nrd1 CID were in slow or intermediate exchange regimes between their free and bound forms relative to the NMR timescale, when titrated with Sen1 NIM, as previously shown for the Trf4-NIM interaction (Tudek et al, 2014), which indicates that the interaction of Nrd1 CID with Sen1 NIM is quite stable. The analysis of changes in chemical shift perturbations (CSP) of Nrd1 CID in the presence of Sen1 NIM and Trf4 NIM peptides suggests that this domain employs the same interaction surface for both NIMs, with only a minor difference in the area of α4-helix and tip of α7-helix (Fig EV2). Click here to expand this figure. Figure EV2. Quantification of chemical shift perturbations (CSP) of Nrd1 CID upon binding to the Trf4 NIM (in red) and Sen1 NIM peptides (in blue)The combined chemical shift perturbations are plotted versus the amino acid residue number. The black boxes indicate α-helices of Nrd1 CID. Download figure Download PowerPoint In order to understand structural basis of the Nrd1–Sen1 interaction, we solved the solution structure of Nrd1 CID (1–153 aa) in complex with Sen1 NIM (Fig 2A; Appendix Table S2). The structure of Nrd1 CID consists of eight α helices in a right-handed superhelical arrangement as previously reported (Vasiljeva et al, 2008; Kubicek et al, 2012; Tudek et al, 2014). The Sen1 NIM peptide is accommodated in the binding pocket of Nrd1 CID in a similar manner to that of Trf4 NIM (Fig 2B and C). The upstream negatively charged part of the Sen1 NIM (D2052–D2053–D2054–E2055–D2056–D2057) interacts with a positively charged region of Nrd1 CID on tips of α1-α2 helices (Fig 2B). Charge-swapping mutations of in this region (K21D, S25D and S28D) resulted in a significant decrease in the binding affinity (Fig 2D; Appendix Table S1), confirming the importance of this region for the interaction. Furthermore, the L20D mutant also diminishes the binding affinity as it perturbs the overall geometry of the α1–α2 loop and thus the positioning of the positively charged residues. The downstream hydrophobic part of Sen1 NIM (Y2058, T2059 and P2060) docks into a hydrophobic pocket of the CID formed by L127, M126 and I130. Y2058 makes a putative H-bond with D70 and R74 of the CID. Sen1 I2062 shows multiple intermolecular contacts in NMR spectra with the aliphatic groups of I130, R133 and S54 side chains. Furthermore, the neighbouring residue S2061 makes a putative H-bond with R74. As a result, these interactions induce the extended conformation of the downstream region of the Sen1 NIM, which contrasts with the formation of the canonical β-turn that was observed in the structures of CTD and NIM peptides bound to CIDs (Meinhart & Cramer, 2004; Becker et al, 2008; Lunde et al, 2010; Kubicek et al, 2012; Tudek et al, 2014). In these complexes, the peptides contain S/NPXX motifs that have a high propensity to form β-turns in which the peptides are locked upon binding to CIDs. In the case of Sen1 NIM, TPSI sequence is predicted as a non-β-turn motif (Singh et al, 2015). Indeed, we found that in the extended conformation the positioning of this downstream motif inside the hydrophobic area is energetically the most favourable. Our structural data suggest that Nrd1 CID is able to accommodate not only peptides with motifs that form β-turns but also peptides in the extended conformation that matches hydrophobicity and H-bonding partners inside the binding groove of the CID. Figure 2. Recognition of Sen1 NIM by Nrd1 CID A. NMR structure of Nrd1 CID bound to Sen1 NIM. The NIM peptide is represented in yellow sticks (only non-hydrogen atoms are shown), and Nrd1 CID is shown as a grey ribbon model. Nrd1 CID residues that form hydrophobic contacts and putative hydrogen bonds to Sen1 NIM peptide are shown in magenta sticks. B. Electrostatic surface representation of Nrd1 CID (electropositive in blue; electronegative in red; neutral in white) with the Sen1 NIM peptide (represented in yellow sticks; only non-hydrogen atoms are shown). The upstream electronegative stretch of Sen1 NIM interacts with an electropositive pocket of Nrd1 CID, while the C-terminal part of the peptide adopts an extended conformation that docks in a hydrophobic pocket of Nrd1 CID. C. Superposition of Nrd1 CID–Sen1 NIM (yellow) and Nrd1 CID–Trf4 NIM (magenta) complexes, displaying only peptide ribbons on the surface of Nrd1 CID. The comparison highlights the extended and β-turn conformations of Sen1 NIM and Trf4 NIM, respectively. D. Scheme showing contacts (putative H-bonds and hydrophobic contacts) and energetics between the Sen1 NIM peptide and Nrd1 CID. Equilibrium binding experiments with the protein mutants were monitored by FA. L20D mutant disrupts the hydrophobic contact with F17 and impairs the overall geometry of the α1-α2 loop that contributes to the interaction with the upstream electronegative stretch of NIM. KD (wild-type Nrd1 CID-Sen1 NIM) equals 1.20 ± 0.02 μM. E. Alignment of RNAPII CTD and CTD mimics (Trf4 NIM, Sen1 NIM and Mpp6 CTD mimic). Blue and grey boxes highlight the upstream electronegative stretches and hydrophobic regions, respectively. Previous structural works reported that S/NPXX motifs form the β-turn conformation (Kubicek et al, 2012; Tudek et al, 2014). Download figure Download PowerPoint A strong interaction between Sen1 and Nrd1-Nab3 is not essential for non-coding transcription termination Because of the importance of the NIM for the interaction between Sen1 and Nrd1-Nab3 heterodimer and its significant conservation among yeast species, we analysed the impact of the NIM deletion on growth and Sen1-mediated transcription termination in vivo. Surprisingly, we found that deletion of the NIM does not affect cell growth and only aggravated the thermosensitive phenotype of a ∆rrp6 mutant, which lacks an exonuclease that plays a major role in degradation of ncRNAs targeted by the NNS complex (Fig EV3A). Click here to expand this figure. Figure EV3. The interaction of Sen1 with Nrd1 and Nab3 is not essential for non-coding transcription termination (related to Fig 3) A. Growth tests of the sen1∆NIM mutant in either a wt or a ∆rrp6 background. B–D. Metagene analyses of RNAseq experiments performed in a ∆rrp6 background in the presence of the wt or the ∆NIM version of Sen1. The profile corresponds to the median coverage (reads per 107 reads mapping at each genomic position) from 0.5 kb upstream to 0.5 kb downstream of the annotated transcription termination site (TTS) of protein-coding genes (B) and CUTs (D) or the 3′ end of the mature snoRNAs (C). Experiments were performed in biological duplicates. E. Deletion of Sen1 C-terminal domain completely abolishes the interaction of Sen1 with Nrd1. Top: scheme of proteins analysed in these experiments. Bottom: CoIP assays using Nrd1-TAP as the bait. Representative gel of one out of two independent experiments. Antibodies used for protein detection are detailed in Appendix Table S3. F. Deletion of Sen1 Cter provokes minor transcription termination defects at typical NNS-dependent non-coding genes. Northern blot assays performed in a ∆rrp6 background. Results correspond to one out of two independent biological replicates. The CAR2 and PHO5 RNAs are detected as a loading controls. Probes used for RNA detection are described in Appendix Table S6. Download figure Download PowerPoint In order to test for the role of Sen1 NIM in non-coding transcription termination, we performed RNAseq transcriptome analyses of ∆rrp6 strains expressing either the wt or the ∆NIM version of Sen1. As expected, metagene analyses did not reveal any significant effect of the NIM deletion at protein-coding genes (Fig EV3B). Surprisingly, we did not observe any major difference in the expression profile of snoRNAs and CUTs in sen1∆NIM (Fig EV3C and D), indicating that deletion of the NIM does not have a general impact on transcription termination at NNS targets. Because in our coimmunoprecipitation experiments, we observed that some minor interaction between Sen1 and Nrd1 persisted after the deletion of Sen1 NIM (Fig 1C); we considered the possibility that this remaining interaction could support sufficient levels of Sen1 recruitment, therefore explaining the weak phenotype of the sen1∆NIM mutant. To investigate this possibility, we deleted the whole C-terminal domain (Cter) of Sen1 downstream of the previously identified nuclear localization signal (Nedea et al, 2008) and analysed the capacity of this mutant (Sen1∆Cter) to interact with Nrd1. Indeed, deletion of the Sen1 Cter did not decrease the protein expression levels but abolished the interaction between Sen1 and Nrd1, indicating that this domain of Sen1 possesses additional surfaces that weakly contribute to the interaction between Sen1 and its partners (Fig EV3E). While this work was under revision, an independent study reported a secondary NIM within the Cter that mediates weak interactions with Nrd1 in vitro, further confirming our observations (Zhang et al, 2019). However, northern blot analyses of several typical NNS targets revealed only minor transcription termination defects in the sen1∆Cter mutant compared to the wt (Fig EV3F). Taken together, our results indicate that the interaction between Sen1 and Nrd1-Nab3 is not a strict requirement for NNS-dependent termination. The integrity of the N-terminal domain of Sen1 is essential for growth and for transcription termination Our observation that the interaction between Sen1 and Nrd1 and Nab3 is not essential for transcription termination is surprising in the light of the model that posits the Nrd1 and Nab3-dependent recruitment of Sen1. Because Sen1 is a low-abundance protein that binds RNA without any sequence specificity, it seems unlikely that it could be adequately
更多查看译文
关键词
transcription,rna,yeast relies
AI 理解论文
溯源树
样例
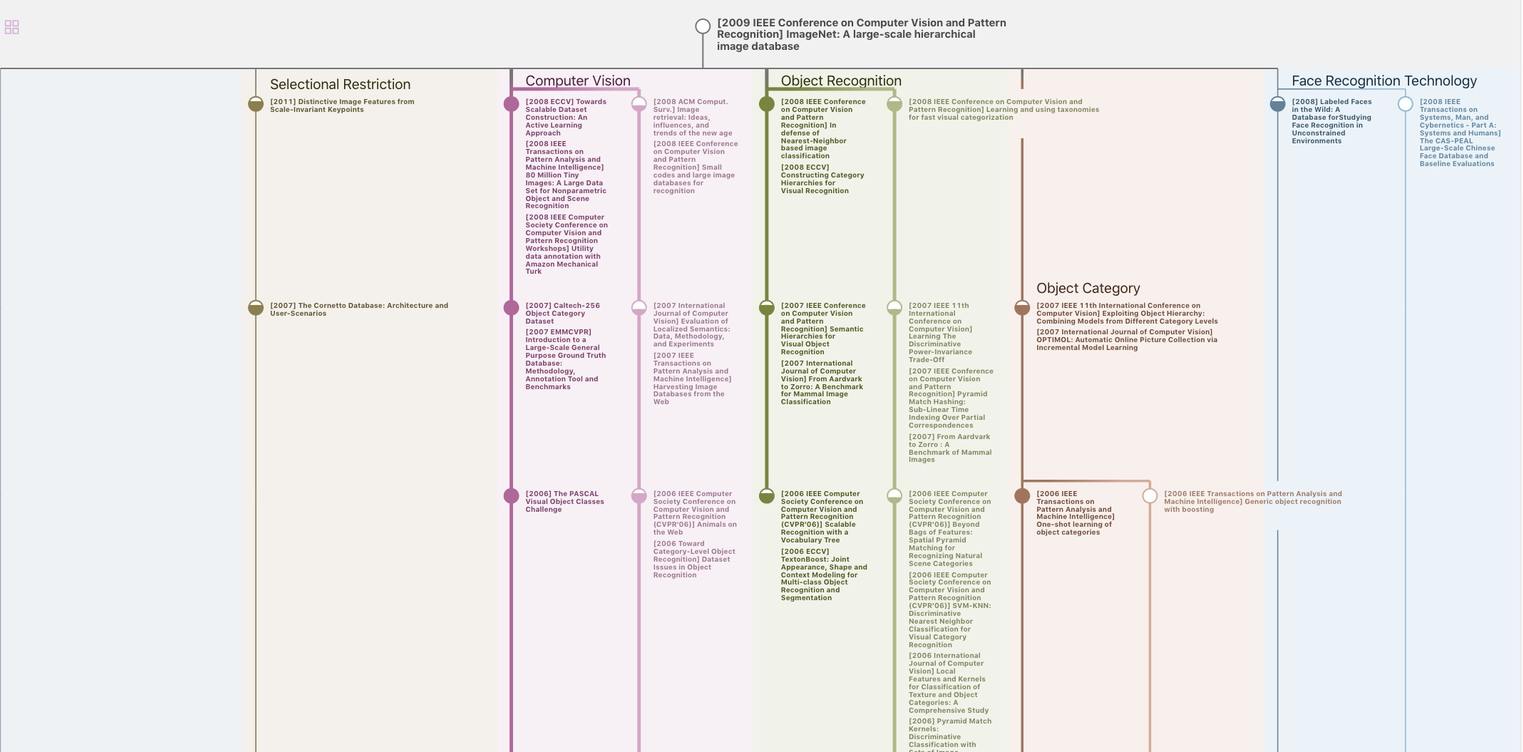
生成溯源树,研究论文发展脉络
Chat Paper
正在生成论文摘要