Osmotic-Enhanced-Photoelectrochemical Hydrogen Production Based on Nanofluidics
CCS Chemistry(2022)
Abstract
Open AccessCCS ChemistryRESEARCH ARTICLES14 Nov 2022Osmotic-Enhanced-Photoelectrochemical Hydrogen Production Based on Nanofluidics Pei Liu†, Xiao-Ya Gao†, Li-Jun Zhang, Weipeng Chen, Yuhao Hu, Xiang-Yu Kong, Xu-Bing Li, Liping Wen, Chen-Ho Tung, Li-Zhu Wu and Lei Jiang Pei Liu† Key Laboratory of Bio-inspired Materials and Interfacial Science, Key Laboratory of Photochemical Conversion and Optoelectronic Materials, Technical Institute of Physics and Chemistry, Chinese Academy of Sciences, Beijing 100190 School of Future Technology, University of Chinese Academy of Sciences, Beijing 100049 †P. Liu and X.-Y. Gao contributed equally to this work.Google Scholar More articles by this author , Xiao-Ya Gao† Key Laboratory of Bio-inspired Materials and Interfacial Science, Key Laboratory of Photochemical Conversion and Optoelectronic Materials, Technical Institute of Physics and Chemistry, Chinese Academy of Sciences, Beijing 100190 School of Future Technology, University of Chinese Academy of Sciences, Beijing 100049 †P. Liu and X.-Y. Gao contributed equally to this work.Google Scholar More articles by this author , Li-Jun Zhang Key Laboratory of Bio-inspired Materials and Interfacial Science, Key Laboratory of Photochemical Conversion and Optoelectronic Materials, Technical Institute of Physics and Chemistry, Chinese Academy of Sciences, Beijing 100190 School of Future Technology, University of Chinese Academy of Sciences, Beijing 100049 Google Scholar More articles by this author , Weipeng Chen Key Laboratory of Bio-inspired Materials and Interfacial Science, Key Laboratory of Photochemical Conversion and Optoelectronic Materials, Technical Institute of Physics and Chemistry, Chinese Academy of Sciences, Beijing 100190 Google Scholar More articles by this author , Yuhao Hu Key Laboratory of Bio-inspired Materials and Interfacial Science, Key Laboratory of Photochemical Conversion and Optoelectronic Materials, Technical Institute of Physics and Chemistry, Chinese Academy of Sciences, Beijing 100190 School of Future Technology, University of Chinese Academy of Sciences, Beijing 100049 Google Scholar More articles by this author , Xiang-Yu Kong *Corresponding authors: E-mail Address: [email protected] E-mail Address: [email protected] E-mail Address: [email protected] E-mail Address: [email protected] E-mail Address: [email protected] Key Laboratory of Bio-inspired Materials and Interfacial Science, Key Laboratory of Photochemical Conversion and Optoelectronic Materials, Technical Institute of Physics and Chemistry, Chinese Academy of Sciences, Beijing 100190 School of Future Technology, University of Chinese Academy of Sciences, Beijing 100049 Google Scholar More articles by this author , Xu-Bing Li *Corresponding authors: E-mail Address: [email protected] E-mail Address: [email protected] E-mail Address: [email protected] E-mail Address: [email protected] E-mail Address: [email protected] Key Laboratory of Bio-inspired Materials and Interfacial Science, Key Laboratory of Photochemical Conversion and Optoelectronic Materials, Technical Institute of Physics and Chemistry, Chinese Academy of Sciences, Beijing 100190 School of Future Technology, University of Chinese Academy of Sciences, Beijing 100049 Google Scholar More articles by this author , Liping Wen *Corresponding authors: E-mail Address: [email protected] E-mail Address: [email protected] E-mail Address: [email protected] E-mail Address: [email protected] E-mail Address: [email protected] Key Laboratory of Bio-inspired Materials and Interfacial Science, Key Laboratory of Photochemical Conversion and Optoelectronic Materials, Technical Institute of Physics and Chemistry, Chinese Academy of Sciences, Beijing 100190 School of Future Technology, University of Chinese Academy of Sciences, Beijing 100049 Google Scholar More articles by this author , Chen-Ho Tung Key Laboratory of Bio-inspired Materials and Interfacial Science, Key Laboratory of Photochemical Conversion and Optoelectronic Materials, Technical Institute of Physics and Chemistry, Chinese Academy of Sciences, Beijing 100190 School of Future Technology, University of Chinese Academy of Sciences, Beijing 100049 Google Scholar More articles by this author , Li-Zhu Wu *Corresponding authors: E-mail Address: [email protected] E-mail Address: [email protected] E-mail Address: [email protected] E-mail Address: [email protected] E-mail Address: [email protected] Key Laboratory of Bio-inspired Materials and Interfacial Science, Key Laboratory of Photochemical Conversion and Optoelectronic Materials, Technical Institute of Physics and Chemistry, Chinese Academy of Sciences, Beijing 100190 School of Future Technology, University of Chinese Academy of Sciences, Beijing 100049 Google Scholar More articles by this author and Lei Jiang *Corresponding authors: E-mail Address: [email protected] E-mail Address: [email protected] E-mail Address: [email protected] E-mail Address: [email protected] E-mail Address: [email protected] Key Laboratory of Bio-inspired Materials and Interfacial Science, Key Laboratory of Photochemical Conversion and Optoelectronic Materials, Technical Institute of Physics and Chemistry, Chinese Academy of Sciences, Beijing 100190 School of Future Technology, University of Chinese Academy of Sciences, Beijing 100049 Google Scholar More articles by this author https://doi.org/10.31635/ccschem.022.202202215 SectionsSupplemental MaterialAboutAbstractPDF ToolsAdd to favoritesDownload CitationsTrack Citations ShareFacebookTwitterLinked InEmail Harvesting clean energy such as solar energy and salinity gradient energy directly from the surrounding environment has attracted great attention. A promising proof-of-concept combination of cation-selective membrane-based osmotic energy with photoelectrochemical-based solar energy has been developed, highlighting the great potential for the direct conversion of osmotic energy to hydrogen energy. With the help of a 50-fold concentration gradient, the MXene–CdSe quantum dots system exhibits the highest photocurrent enhancement ratio (ΔIL-H/ΔIL-L), and the hydrogen production is increased by about 33% at a bias of 0 V versus reversible hydrogen electrode. Directly converting osmotic energy and solar energy into hydrogen energy suggests the possibility of coupling osmotic energy with other renewable energy sources. Download figure Download PowerPoint Introduction The rapid consumption of fossil fuels and the ensuing environmental problems have forced the exploration of low-cost, sustainable, and ecofriendly energy sources.1–3 Renewable energy generated from solar, wind, biomass, hydro, ocean, and heat in the earth’s crust has gained increasing attention.3–5 Ocean energy has become an important part of the development of new energy due to its large storage capacity and diverse development methods; particularly, osmotic energy from the salinity gradient between ocean water and river water, also known as blue energy, has recently been identified as a promising source of clean energy.6–9 Nanofluidics combine strong confinement and surface-charge effects at the nanoscale, which contribute to novel ion transport properties including excellent selectivity and high flux.10 Nanofluidic channels with tailored ion transport dynamics enable high-performance reverse electrodialysis to efficiently harvest renewable osmotic energy. Therefore, various symmetric and asymmetric nanofluidics using materials of different dimensions have been developed to finely control ion transport behaviors to capture osmotic energy.11–14 However, almost all osmotic energy is converted into electrical energy and then for further power output or external resistance supply (see Supporting Information Figure S1). Solar energy transformation via photoelectrochemical (PEC) hydrogen generation is another important pathway of clean energy generation.15–19 With the excitation of suitable visible light, hydrogen production by photogenerated electrons and oxygen generation by photogenerated holes can be realized with a rationally designed PEC system, which can convert solar energy into chemical energy. However, there are few studies reported on enhancing PEC hydrogen generation with the help of osmotic energy.11 In contrast, PEC systems demand an additional bias voltage to assist directional transmission of charge and enhance carrier separation,17,20,21 whereas osmotic energy and internal concentration gradient can generate an extra potential. The introduction of osmotic energy might contribute to the enhancement of PEC water splitting. Additionally, the membrane separates the electrolyte between anode and cathode to avoid the side reaction of products and separately collects the products generated on the electrode surface. Therefore, it would be inspiring for simultaneously storing solar energy and osmotic energy in chemical bonds. With this in mind, we embarked on the design of a system that could directly convert osmotic energy and solar energy to chemical products, such as H2 and O2 (Figure 1). Among various materials, semiconductor quantum dots (QDs) have been widely used for artificial photosynthetic hydrogen production because of their unique properties such as large extinction coefficients, abundant surface sites, and tunable band position. Moreover, QDs can evolve H2 gas at the exposed metal sites in the absence of external cocatalysts. Therefore, we used colloidal QDs in this system.22 Under light irradiation, the QDs sensitized photocathode would absorb photons and generate the photoinduced electron-hole pairs. The photogenerated holes transfer from the valence band of QDs to the counter electrode (Pt) to participate in the oxidation reactions, and the photogenerated electrons could migrate to the electrode surface followed by proton reduction, that is, H2 generation. With the participation of the concentration gradient and the cation selectivity membrane, the directional migration of Na+ across the membrane would occur, thus leading to the accumulation of cations on the photocathode side. To maintain the electrical neutrality, cations need to be consumed and the reduction of protons on the photocathode would be facilitated,23 thereby achieving osmotic-enhanced PEC hydrogen production. In brief, this process could directly convert osmotic energy into hydrogen energy and further promote hydrogen production. Figure 1 | Coupling of osmotic-energy conversion and the PEC hydrogen production. Under light irradiation, the QD-sensitized photocathode would absorb photons and generate the photoinduced charge carriers, electron–hole pairs. The holes transfer from the QDs to the counter electrode (Pt) through an external circuit and oxidize water to generate O2 while the remaining electrons are used to reduce protons into H2, simultaneously. Meanwhile, directional migration of Na+ across the cation selective membrane driven by concentration difference leads to the accumulation of cations in the low-concentration side. To maintain electrical neutrality, the reduction of protons on the photocathode is enhanced, thereby achieving osmotic-enhanced PEC hydrogen production. This system realizes the direct conversion of solar energy and osmotic energy to hydrogen energy. Download figure Download PowerPoint Here, we provide a demonstration of osmotic enhanced PEC hydrogen production based on nanofluidics (Figure 1). Osmotic energy is introduced into the PEC hydrogen production system by using various cation selective membranes made of materials with different dimensions. Upon visible-light irradiation, the photocurrent of the PEC system with the concentration gradient is significantly enhanced compared with that without the concentration gradient. The MXene–CdSe QDs system shows the highest photocurrent enhancement ratio (ΔIL-H/ΔIL-L) and realizes an approximately 33% increase in hydrogen production at a bias of 0 V versus reversible hydrogen electrode (RHE) while maintaining a stable Faraday efficiency, which does not require any sacrificial agents or external cocatalyst. The intended system proposes and verifies the concept of osmotic-enhanced PEC hydrogen production for the first time. Furthermore, this work has realized the direct conversion of osmotic energy to other renewable energy sources such as hydrogen energy. Experimental Methods Fabrication of cellulose nanofiber, MXene, acrylic acid, graphene oxide, and molybdenum disulfide membrane Cellulose nanofiber membrane 2,2,6,6-tetramethylpiperidine-1-oxyl (TEMPO)-oxidized cellulose nanofiber (CNF) gel (1 wt %) was purchased from Woodelfbio Cellulose Co., Ltd. (Tianjin, China). A certain quantity of CNF gel was dispersed in deionized (DI) water by ultrasound to obtain a dispersion of 1 mg/mL; then, 30 mL CNF dispersion was filtered on polycarbonate (PC, pore size ∼200 nm) and self-assembled into a free-standing membrane. Finally, the CNF membrane was peeled off easily after drying in air. MXene membrane First, 0.66 g LiF was slowly added to 10 mL of 9 M HCl solution and stirred for 5 min. Then, 1 g MAX Ti3AlC2 powders (98%, 200 mesh, purchased from Forsman Scientific Co., Ltd., Beijing, China) were added and stirred at 800 rpm for 24 h at 35 °C. After etching, the mixture was centrifuged and washed with DI water at 3500 r.p.m. for 5 min each until a pH ∼6. The settled powders were dried for at least 12 h and collected. A certain quantity of Ti3C2Tx was dissolved into dimethyl sulfoxide and then magnetically stirred at room temperature for 2 h to delaminate. The intercalated-Ti3C2Tx was then dispersed in DI water with concentration of 1 mg/mL, followed by sonication under flowing argon for 10 min, and then centrifugation for 1 h at 3500 rpm. The MXene supernatant was obtained, and then 500 μL MXene dispersion was filtered on anodic aluminum oxide (AAO, pore size 110–150 nm) to obtain the 2D MXene membrane. Acrylic acid membrane The precursor solution for preparing acrylic acid (AAc) hydrogel membrane was composed of 2 g of AAc, 40 mg of N,N′-methylenebis(acrylamide), 0.1 g of methyl methacrylate, 10 μL of 2,2-diethoxyacetophenone, and 7.9 g of DI water. First, the precursor solution was poured on PC film for 5 min to ensure the solution penetrated the channel and filled the space. The excess precursor solution was removed by filter paper. After the photopolymerization under UV irradiation (PL-LED100, 365 nm, Perfectlight Technology Co., Ltd., Beijing, China), the hybrid membrane was obtained. Before testing, all the hydrogel hybrid membranes were soaked in water for 24 h (with a fresh water change every 6 h) to ensure the removal of excess monomers and stored in water for later use. Graphene oxide and molybdenum disulfide membrane Graphene oxide (GO) and molybdenum disulfide (MoS2) dispersions were purchased from XFNANOMaterials Tech Co., Ltd. (Nanjing, China). The GO or MoS2 dispersions (500 μL) were filtered on an AAO (pore size 110–150 nm) to obtain the 2D membrane. Fabrication of QDs/NiO/fluorine-doped tin oxide electrode Fluorine-doped tin oxide/NiO electrode The NiO precursor solution was first prepared by reflux of 6 mmol of Ni(Ac)2·4H2O, 6 mmol of ethanolamine, and 20 mL of 2-methoxyethanol at 70 °C for 1 h. Fluorine-doped tin oxide (FTO) glasses (2 cm × 2 cm) were sonicated respectively for 30 min in detergent solution, water, and ethanol to remove contaminant. The NiO precursor solution (180 μL) was printed on the precleaned FTO glass using the spin-coating method (3000 r.p.m., 30 s), followed by being sintered at 400 °C for 60 min in a muffle furnace with the heating rate of 8 °C/min. Finally, FTO/NiO electrode was obtained by natural cooling. QDs/NiO/FTO electrode QDs (5 mL) was precipitated by adding 25 μL of HCl (1 M), followed by centrifuging and washing with water twice. The precipitate was dispersed in 800 μL of water and sonicated for 30 min. The dispersed solution (160 μL) was dropped on the surface (1 cm × 1 cm) of the FTO/NiO electrode (2 cm × 1 cm), and the electrode was dried naturally. Finally, the electrode was calcined in a tube furnace under argon atmosphere for 20 min at 150 °C with the heating rate of 5 °C/min. Electrical measurements The ionic transport properties and osmotic energy conversion tests of cation selectivity membranes were performed with a Keithley 6487 semiconductor picoammeter (Keithley Instruments, Cleveland, OH). The membranes were mounted between a two-compartment conductivity cell that contained about 3.0 mL testing solution (see Supporting Information Figure S2). For the ionic transport measurement, KCl solutions from 0.1 μM to 1 M were used to fill two chambers. Homemade Ag/AgCl electrodes were used to apply a transmembrane potential across the membrane. For the energy conversion measurement, concentrated and diluted solutions were filled into two sides of the membrane. Homemade Ag/AgCl electrodes were used to collect the osmotic energy. The short-circuit current ISC (the current corresponding to zero external bias) and open-circuit potential VOC (the potential corresponding to the zero current) were obtained from the I–V measurements. In these measurements, the effective experimental area was about 3.14 mm2. All the measurement were conducted under pH 6. PEC H2 evolution The schematic diagram of the PEC device is shown in Figure 5a. The cation selective membrane is fixed in the middle of the two cells. The prepared electrode acted as the working electrode, Ag/AgCl (saturated KCl) as the reference electrode, and platinum as the counter electrode. Na2SO4 aqueous solution (80 mL) built the concentration gradient and acted as the electrolyte. After sealing, the electrolyte solution was bubbled with inert argon gas for 20 min to remove oxygen. The I–t measurement was tested by an electrochemical workstation with blue light-emitting diodes (LEDs) irradiating the working electrode. The produced hydrogen was detected by gas chromatograph (GC) with CH4 as an internal standard. In the isotope labeling experiments, the water on the counter electrode side was replaced with 1:1 H216O and H218O, with other conditions unchanged. 18O2 was detected by gas chromatography-mass spectrometry (GC-MS). All light irradiation experiments were carried out at room temperature with ambient temperature condensate. Results and Discussion One-dimensional (1D) nanofibers (CNF), two-dimensional (2D) nanosheets (MXene), and three-dimensional (3D) hydrogels (AAc) were selected to build cation selective membranes (Figure 2a).24–26 The length and diameter of the CNF were in the range of micrometer and nanometer scale, respectively (see Supporting Information Figure S3), which rendered the decent stacking of the 1D nanofibers to form the nanofluidic membrane (Figure 2a-i). Ti3AlC2 was chosen to fabricate MXene nanosheets as reported in our previous work.25,27 The obtained 2D MXene nanosheets could be reassembled through vacuum assisted filtration to get a flexible thin membrane attached on the AAO substrate whose channel size was within the range of 110–150 nm. The cross-sectional scanning electron microscopy (SEM, Supporting Information Note 3) image of the MXene membrane showed an ordered lamellar structure (Fig. 2a-ii). 3D hydrogel networks were constructed through radical initiated polymerization in the channels of the PC film. As shown in Fig. 2a-iii, the channels of the PC film were fully filled with 3D hydrogel after hybridization. Moreover, X-ray photoelectron spectroscopy (XPS) was used to further study the functional groups of the three membranes (see Supporting Information Figure S4). The cation selectivity of CNF and AAc membranes benefited from the carboxyl groups (–COOH). Furthermore, in the high-resolution XPS spectrum of the MXene membrane, the O1s spectrum indicated four components: O–Ti, O–Ti/OH, O–C/OH, and H2O. These surface groups imparted the MXene nanosheets a negatively charged surface and cation selectivity. Figure 2 | Characterization and osmotic-energy conversion behavior of the three cation-selective membranes made from materials of different dimensions: 1D CNF, 2D MXene, and 3D AAc hydrogels. (a) Cross-sectional SEM and the corresponding schematics of the structure of the three membranes: (i) 1D CNF, (ii) 2D MXene, and (iii) 3D AAc hydrogels. (b) Ion conductance of the membranes versus KCl concentration, showing a typical charge-governed ion transport behavior. (c) ISC and (d) VOC under different concentration gradient (NaCl). The low-concentration side is fixed at 0.01 M. Download figure Download PowerPoint The ionic transport properties of the three cation selectivity membranes were characterized under various ionic concentrations. Supporting Information Figure S5a shows the I–V characteristics of various membranes under symmetric electrolyte condition (0.01 M KCl, pH ∼7). The MXene membrane exhibited the smallest current value at −2 V among the three materials (See Supporting Information Figure S5b) due to its sub-nanometer-sized ion channels. Moreover, transmembrane ionic conductance was significantly affected by electrolyte concentration (Figure 2b). These conductance curves gradually deviated from the volume value (gray dotted line) and showed saturation for low salt concentration, indicating the typical surface-charge-controlled ion transport process across the membrane.28 Next, the osmotic energy conversion performance was demonstrated by using NaCl with various concentration gradients applied across the composite membrane. The short-circuit current (ISC, current at 0 V) and open-circuit potential (VOC, potential corresponding to zero current) are summarized in Figure 2c,d. As mentioned above, the ion channels of the MXene membrane were sub-nanometer-sized so that the corresponding ISC and VOC were lower than those of CNF and AAc. The high cation selectivity of the AAc membrane owing to the space charge from the carboxylic group of the hydrogel 3D network led to the higher osmotic energy conversion performance.24,29 Then, osmotic enhanced PEC hydrogen production was investigated based on the above three cation selectivity membranes. First, the PEC hydrogen generation system was constructed with a sensitized photocathode (FTO/NiO/QDs), QDs as the photocatalyst unit, and p-type NiO as the hole transfer layer. Three kinds of QDs were selected to fabricate the photocathodes in this process ( Supporting Information Note 1).30–32 Dynamic light scattering ( Supporting Information Figure S6) indicated the stable colloidal property and good dispersion of mercaptopropionic acid (MPA) stabilized CdSe QDs, CdS QDs, and CdSe/CdS core-shell QDs in water. Figure 3a displays the high-resolution transmission electron microscopy (TEM) images of CdSe QDs, CdS QDs, and CdSe/CdS core-shell QDs. Upon light irradiation, the QDs sensitized photocathode would generate the photoinduced electron-hole pairs. The photoinduced holes transferred from QDs to the counter electrode (Pt) through an external circuit and oxidized water to generate O2 while the electrons were involved in simultaneous hydrogen production. UV–vis absorption spectra of CdSe, CdS, and CdSe/CdS QDs are shown in Figure 3b, corresponding to the first exciton absorption peak of 438, 420, and 468 nm, respectively, indicating their good visible-light response. Figure 3 | Osmotic-enhanced photocurrent responses to on–off illumination. (a) Schematic illustration of the photocathode and the migration of photogenerated excitons at the interfaces. The corresponding high-resolution TEM images of CdSe QDs, CdS QDs, and CdSe/CdS QDs are shown on the right. (b) UV–vis spectra and photographs of the CdSe QDs, CdS QDs, and CdSe/CdS QDs. (c) The difference between the photocurrent and the dark-current (IPhoto − IDark) with (L-H: 0.01 M/0.5 M Na2SO4) or without concentration gradient (L-L: 0.01 M/0.01 M Na2SO4) at a bias of 0 V versus RHE. (d) Photocurrent enhancement ratio (ΔIL-H/ΔIL-L) of different systems. Download figure Download PowerPoint For concept study, osmotic-enhanced PEC hydrogen generation performance was investigated in a three-electrode H-shape cell separated by cation selective membranes with QDs-sensitized photocathode as the working electrode, platinum (Pt) as the counter electrode, and Ag/AgCl as the reference electrode ( Supporting Information Figure S7, see details in SI). Oxygen-free Na2SO4 aqueous solution with certain concentration acted as the electrolyte. Upon the same or different electrolyte concentration for both sides of the membrane, the FTO/NiO/QDs photocathode33–35 initiated a cathodic photocurrent with a bias of 0 V versus RHE under blue LEDs (λ = 450 nm) irradiation, suggesting hole transfer to counter electrode and proton reduction on the surface of the FTO/NiO/QDs photocathode ( Supporting Information Figures S8–S10). The introduction of concentration gradient and cation selectivity membrane led to the accumulation of cations on the photocathode side and further promoted the consumption of protons, achieving an enhancement of photocurrent ( Supporting Information Figures S8–S10). We note that the introduction of AAO, working as a supporting layer, had basically no effect on the enhancement of photocurrent in the AAO/MXene composite membrane ( Supporting Information Figure S11). Additionally, the difference between photocurrent and dark-current (IPhoto − IDark) with (L-H: 0.01 M/0.5 M Na2SO4) or without concentration gradient (L-L: 0.01 M/0.01 M Na2SO4) are summarized in Figure 3c (at a bias of 0 V vs RHE). The IPhoto − IDark under the 50-fold concentration gradient was significantly higher than that without concentration gradient for all systems. By examining a range of cation selective membranes (including CNF, MXene, and AAc) and three kinds of QDs (CdSe, CdS, and CdSe/CdS QDs), the maximum photocurrent enhancement ratio (ΔIL-H/ΔIL-L) was realized by osmotic promotion with MXene as the cation-selective membrane, and CdSe QDs as the photocatalyst (Figure 3d). Compared with nanofluids composed of 1D and 3D materials, ion-selective membranes constructed from 2D-layered materials show ordered ion transport pathways and thus have lower ion transport resistance. Moreover, different cation selective membranes provide a different built-in electric field due to different cation selectivity, ion flux, and ion migration rates, and these factors could also lead to different diffusion kinetics of sodium ions within the membrane. The photocurrent maximally enhanced for the MXene-CdSe system might be ascribed to the balance between the diffusion kinetics of sodium ions within the membrane and the kinetics of the redox reactions. The whole linear sweep voltammetry (LSV) curve was measured ( Supporting Information Figure S12), and the most positive shift for the MXene/CdSe system suggests it is the best combination. Compared with the condition without the concentration gradient, the photocurrent in the presence of concentration gradient exhibits a nonlinear increase with the negative shift of the potential because the average salinity difference between catholyte and anolyte gradually decreases, thus leading to the lower open-circuit voltage. Furthermore, compared with conditions lacking the concentration gradient, MXene/CdSe exhibits a larger cathodic current, more positive onset potential, and better PEC activity within the whole potential range, which confirms that the concentration gradient could indeed provide potential to increase PEC water splitting. According to the LSV curve, different cationic selective membranes exhibit different PEC activities with the same CdSe QDs photocathode. Based on Faraday’s law of electrolysis, the number of electrons transferred by the chemical reaction that occurs at the photocathode or photoanode is equal to the quantity of electricity passed through the entire circuit. The PEC water splitting reaction is driven by the photo potential, the extra bias, and the osmotic potential provided by the concentration gradient. With the same photoelectrode and the reaction condition (pH, concentration of Na2SO4), the main factor leading to the different reaction kinetics is the different osmotic energy, which leads to different diffusion kinetics of sodium ions within the membrane and produces a different built-in electric field. Furthermore, to prove the universality of t
MoreTranslated text
Key words
hydrogen,osmotic-enhanced-photoelectrochemical
AI Read Science
Must-Reading Tree
Example
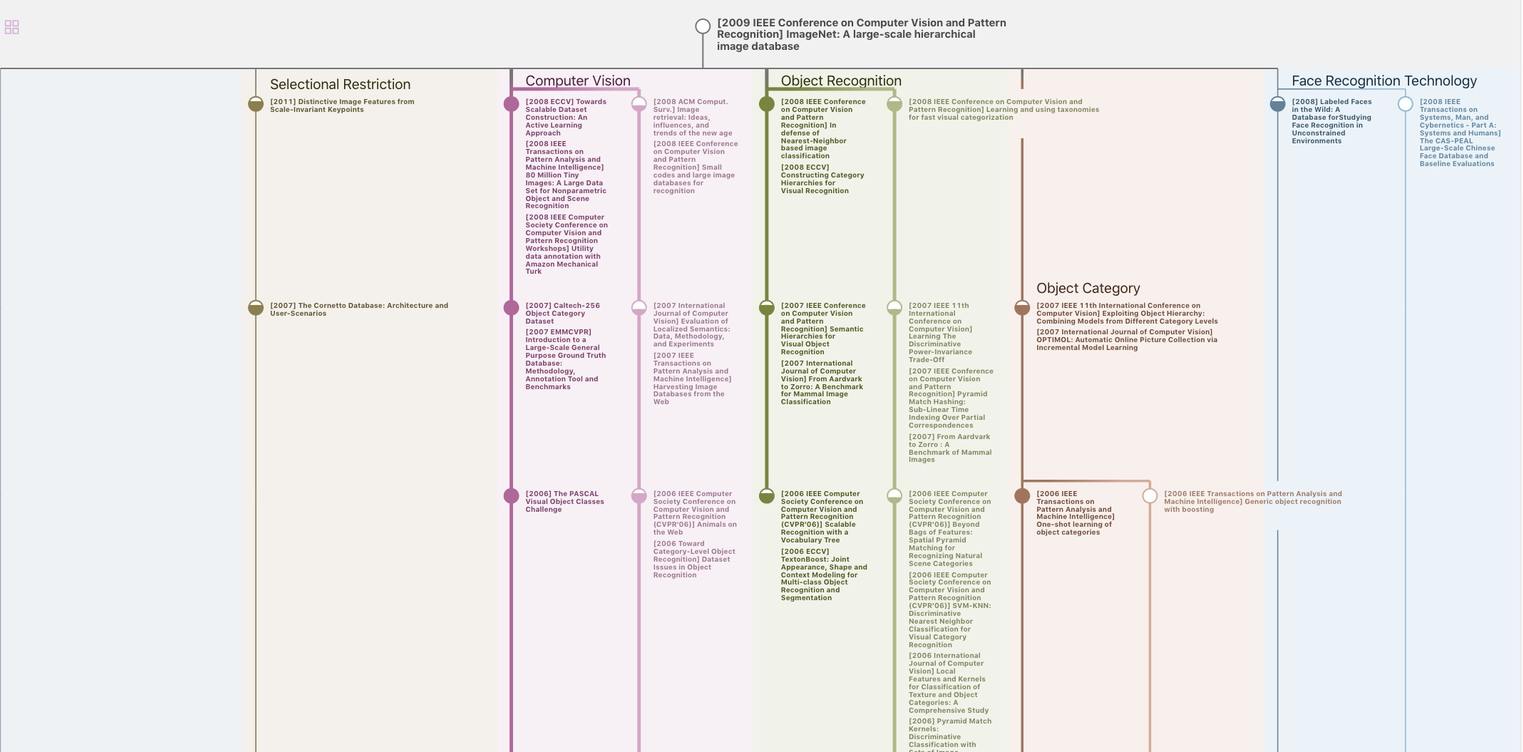
Generate MRT to find the research sequence of this paper
Chat Paper
Summary is being generated by the instructions you defined