Bacterial killing by complement requires membrane attack complex formation via surface‐bound C5 convertases
The EMBO Journal(2019)
摘要
Article14 January 2019Open Access Transparent process Bacterial killing by complement requires membrane attack complex formation via surface-bound C5 convertases Dani AC Heesterbeek Dani AC Heesterbeek Department of Medical Microbiology, University Medical Center Utrecht, Utrecht University, Utrecht, The Netherlands Search for more papers by this author Bart W Bardoel Bart W Bardoel Department of Medical Microbiology, University Medical Center Utrecht, Utrecht University, Utrecht, The Netherlands Search for more papers by this author Edward S Parsons Edward S Parsons London Centre for Nanotechnology, University College London, London, UK Search for more papers by this author Isabel Bennett Isabel Bennett London Centre for Nanotechnology, University College London, London, UK Search for more papers by this author Maartje Ruyken Maartje Ruyken Department of Medical Microbiology, University Medical Center Utrecht, Utrecht University, Utrecht, The Netherlands Search for more papers by this author Dennis J Doorduijn Dennis J Doorduijn Department of Medical Microbiology, University Medical Center Utrecht, Utrecht University, Utrecht, The Netherlands Search for more papers by this author Ronald D Gorham Jr Ronald D Gorham Jr Department of Medical Microbiology, University Medical Center Utrecht, Utrecht University, Utrecht, The Netherlands Search for more papers by this author Evelien TM Berends Evelien TM Berends Department of Medical Microbiology, University Medical Center Utrecht, Utrecht University, Utrecht, The Netherlands Search for more papers by this author Alice LB Pyne Alice LB Pyne London Centre for Nanotechnology, University College London, London, UK Search for more papers by this author Bart W Hoogenboom Bart W Hoogenboom orcid.org/0000-0002-8882-4324 London Centre for Nanotechnology, University College London, London, UK Department of Physics and Astronomy, University College London, London, UK Search for more papers by this author Suzan HM Rooijakkers Corresponding Author Suzan HM Rooijakkers [email protected] orcid.org/0000-0003-4102-0377 Department of Medical Microbiology, University Medical Center Utrecht, Utrecht University, Utrecht, The Netherlands Search for more papers by this author Dani AC Heesterbeek Dani AC Heesterbeek Department of Medical Microbiology, University Medical Center Utrecht, Utrecht University, Utrecht, The Netherlands Search for more papers by this author Bart W Bardoel Bart W Bardoel Department of Medical Microbiology, University Medical Center Utrecht, Utrecht University, Utrecht, The Netherlands Search for more papers by this author Edward S Parsons Edward S Parsons London Centre for Nanotechnology, University College London, London, UK Search for more papers by this author Isabel Bennett Isabel Bennett London Centre for Nanotechnology, University College London, London, UK Search for more papers by this author Maartje Ruyken Maartje Ruyken Department of Medical Microbiology, University Medical Center Utrecht, Utrecht University, Utrecht, The Netherlands Search for more papers by this author Dennis J Doorduijn Dennis J Doorduijn Department of Medical Microbiology, University Medical Center Utrecht, Utrecht University, Utrecht, The Netherlands Search for more papers by this author Ronald D Gorham Jr Ronald D Gorham Jr Department of Medical Microbiology, University Medical Center Utrecht, Utrecht University, Utrecht, The Netherlands Search for more papers by this author Evelien TM Berends Evelien TM Berends Department of Medical Microbiology, University Medical Center Utrecht, Utrecht University, Utrecht, The Netherlands Search for more papers by this author Alice LB Pyne Alice LB Pyne London Centre for Nanotechnology, University College London, London, UK Search for more papers by this author Bart W Hoogenboom Bart W Hoogenboom orcid.org/0000-0002-8882-4324 London Centre for Nanotechnology, University College London, London, UK Department of Physics and Astronomy, University College London, London, UK Search for more papers by this author Suzan HM Rooijakkers Corresponding Author Suzan HM Rooijakkers [email protected] orcid.org/0000-0003-4102-0377 Department of Medical Microbiology, University Medical Center Utrecht, Utrecht University, Utrecht, The Netherlands Search for more papers by this author Author Information Dani AC Heesterbeek1,‡, Bart W Bardoel1,‡, Edward S Parsons2, Isabel Bennett2, Maartje Ruyken1, Dennis J Doorduijn1, Ronald D Gorham1, Evelien TM Berends1, Alice LB Pyne2, Bart W Hoogenboom2,3 and Suzan HM Rooijakkers *,1 1Department of Medical Microbiology, University Medical Center Utrecht, Utrecht University, Utrecht, The Netherlands 2London Centre for Nanotechnology, University College London, London, UK 3Department of Physics and Astronomy, University College London, London, UK ‡These authors contributed equally to this work *Corresponding author. Tel: +31 88 75 504 86; E-mail: [email protected] The EMBO Journal (2019)38:e99852https://doi.org/10.15252/embj.201899852 PDFDownload PDF of article text and main figures. Peer ReviewDownload a summary of the editorial decision process including editorial decision letters, reviewer comments and author responses to feedback. ToolsAdd to favoritesDownload CitationsTrack CitationsPermissions ShareFacebookTwitterLinked InMendeleyWechatReddit Figures & Info Abstract The immune system kills bacteria by the formation of lytic membrane attack complexes (MACs), triggered when complement enzymes cleave C5. At present, it is not understood how the MAC perturbs the composite cell envelope of Gram-negative bacteria. Here, we show that the role of C5 convertase enzymes in MAC assembly extends beyond the cleavage of C5 into the MAC precursor C5b. Although purified MAC complexes generated from preassembled C5b6 perforate artificial lipid membranes and mammalian cells, these components lack bactericidal activity. In order to permeabilize both the bacterial outer and inner membrane and thus kill a bacterium, MACs need to be assembled locally by the C5 convertase enzymes. Our data indicate that C5b6 rapidly loses the capacity to form bactericidal pores; therefore, bacterial killing requires both in situ conversion of C5 and immediate insertion of C5b67 into the membrane. Using flow cytometry and atomic force microscopy, we show that local assembly of C5b6 at the bacterial surface is required for the efficient insertion of MAC pores into bacterial membranes. These studies provide basic molecular insights into MAC assembly and bacterial killing by the immune system. Synopsis The complement is an essential part of the immune system that kills target cells via lytic membrane attack complex (MAC) pores. Complement C5 convertases regulate local assembly of MAC pores and their insertion into bacterial membranes to trigger bacterial killing. Purified MAC components generated from preassembled C5b6 perforate artificial lipid membranes but lack bactericidal activity. Local assembly of C5b6 by a C5 convertase is essential to form bactericidal MAC pores that damage both bacterial membranes. Local assembly of C5b6 at the bacterial surface is required for the efficient insertion of MAC pores into bacterial membranes. Introduction Membrane attack complex (MAC) formation is an evolutionarily conserved immune mechanism to kill bacteria and altered self-cells. It results from activation of the complement cascade (present in blood and most bodily fluids; Kang et al, 2009; Ricklin et al, 2010), when newly formed C5b6 complexes bind C7, C8, and multiple C9 molecules to build hetero-oligomeric MAC pores into target cell membranes. The MAC has an essential role in human immune protection against Gram-negative bacteria; this is evident from recurrent infections in patients lacking MAC activity due to genetic deficiencies (Ram et al, 2010; Turley et al, 2015) or due to treatment with complement-inhibitory drugs (Konar & Granoff, 2017; McNamara et al, 2017; Ricklin et al, 2017). Since MAC-dependent cell lysis can be specifically triggered via antibodies, this killing mechanism is also exploited for therapeutic development of antibodies that target cancer cells or drug-resistant bacterial infections (Szijártó et al, 2015; de Jong et al, 2016). Despite its crucial role in immunity, it is currently not understood how the MAC kills bacteria. In vivo, the MAC is generated via an enzymatic chain reaction on the target cell surface (Ricklin et al, 2010; Berends et al, 2014). Following recognition of a foreign cell via antibodies or pattern recognition molecules, proteins of the complement system (Ricklin et al, 2010; Ugurlar et al, 2018) rapidly organize into a proteolytic cascade that eventually results in cleavage—by C5 convertase enzymes—of precursor C5 into the anaphylatoxin C5a and C5b (Gros et al, 2008; Ricklin et al, 2010); C5b initiates the assembly of the MAC (C5b-9; Hadders et al, 2012; Serna et al, 2016; Sharp et al, 2016; Bayly-Jones et al, 2017). Nascent C5b by itself is labile, but forms stable C5b6 complexes by rapid association with C6 (Cooper & Müller-Eberhard, 1970; Hadders et al, 2012). Next, C5b6 complexes bind C7, which changes conformation to expose a hydrophobic domain that renders the complex lipophilic (Preissner et al, 1985). Once C5b-7 is bound to the target membrane, its assembly with C8 and 18 copies of C9 results in the formation of the MAC (Bayly-Jones et al, 2017; preprint: Parsons et al, 2018). Recent in vitro structural studies (Serna et al, 2016; Sharp et al, 2016; Menny et al, 2018; preprint: Parsons et al, 2018) have revealed detailed information on how MAC proteins form toroid-shaped pores with an inner diameter of 10 nm, spanning a single phospholipid (bilayer) membrane. Despite these structural insights, it remains unclear how these pores can kill Gram-negative bacteria. Since the cytoplasmic membrane of Gram-negative bacteria is physically protected by a peptidoglycan layer and an outer membrane (Silhavy et al, 2010), it is unclear how MAC pores, with a transmembrane region of < 10 nm (Serna et al, 2016; Sharp et al, 2016), can perturb this composite cell wall. Furthermore, it is not known if MAC can perturb both membranes and if other serum components, such as the peptidoglycan-degrading enzyme lysozyme (Wright & Levine, 1981), are required to kill a bacterium. In this paper, we demonstrate that although the purified MAC components (C5b-9) form pores in artificial lipid membranes (Serna et al, 2016; Sharp et al, 2016), they require additional complement components to be bactericidal. Specifically, we find that MAC-dependent killing critically depends on the prior labeling of the bacterial surface with C5 convertase enzymes. Using novel membrane perturbation analyses and atomic force microscopy, we here show that in situ cleavage of C5 by convertases at the microbial surface and immediate insertion of newly formed C5b67 complexes into the membrane is essential for the MAC to perturb both bacterial membranes and to be bactericidal. Our data highlight a critical role for complement activation mechanisms at the cell surface to induce bacterial killing. Results MAC in serum perturbs both the outer and inner membrane of Gram-negative bacteria To better understand bacterial killing by the MAC, we developed a flow cytometry-based approach that can distinguish between outer and inner membrane perforation in Gram-negative bacteria following exposure to human serum, which contains all complement proteins and has potent bactericidal activity against Gram-negative bacteria (Berends et al, 2014). Outer membrane integrity was monitored by measuring release of mCherry from the periplasmic space of genetically engineered E. coli MG1655 cells (Fig 1A and B). Inner membrane integrity was monitored by detecting release of cytosolic Green Fluorescent Protein (GFP) or by the influx of small molecule DNA dyes (Lebaron et al, 1998). Upon exposure of these cells to human serum, the periplasmic mCherry signal decreased in the entire population, indicating permeabilization of the outer membrane (Figs 1C and EV1A). Although cytosolic GFP signals remained constant, human serum induced effective passage of small DNA dyes (Figs 1C and EV1A). Figure 1. MAC in serum perturbs both the outer and inner membrane of Gram-negative bacteria A. Schematic representation of engineered perimCherry/cytoGFP E. coli cells that express mCherry in the periplasmic space (between the outer and inner membrane) and GFP in the cytosol. B. Structured illumination microscopy image of perimCherry/cytoGFP E. coli confirming localization of mCherry (red) in the periplasm and GFP (green) in the cytosol. Scale bar = 3 μm. C. Outer membrane damage (mCherry intensity) and inner membrane damage (% Sytox positive) of perimCherry/cytoGFP E. coli bacteria exposed to (different concentrations of) human serum. Inner membrane damage correlates with killing (samples where bacteria are killed are indicated with gray shadings and a cross, see CFU data in Fig EV1B). D, E. (D) Serum-induced inner membrane damage (% Sytox positive) and (E) killing (CFU/ml) of different Gram-negative strains depends on MAC components C5 and C8, but not on lysozyme (10% serum). Dotted line represents the detection limit of the assay. Data information: The cfu/ml (E) and Sytox measurements (D) of “Buffer”, “Serum”, “ΔC5”, “ΔC8”, “Δlysozym”, and “C5b6MAC” were all generated from the same experiment. (C–E) Data represent mean ± SD of 3 independent experiments. (D, E) Statistical analysis was done using a ratio paired two-tailed t-test and displayed only when significant as *P ≤ 0.05, **P ≤ 0.01, ***P ≤ 0.001, or ****P ≤ 0.0001. Download figure Download PowerPoint Click here to expand this figure. Figure EV1. Serum-induced inner membrane damage is essential for bacterial killing Representative flow cytometry plots of perimCherry/cytoGFP E. coli after 30 min of exposure to buffer or 10% human serum. Bacterial viability (via colony enumeration on agar plates) of perimCherry/cytoGFP E. coli exposed to a concentration range of serum (samples identical to Fig 1C). Successful depletion of serum from lysozyme (lysozyme-specific ELISA; black line), but sustained complement activity (CH50; red). Data information: (B, C) Data represent mean ± SD of 3 independent experiments. (B) Statistical analysis was done using a ratio paired two-tailed t-test and displayed only when significant as *P≤0.05. Download figure Download PowerPoint By carefully titrating concentrations of serum (and thus complement components), we found that the influx of DNA dyes, but not mCherry release, strongly correlated with bacterial cell death (Figs 1C and EV1B). Also, in two wild-type E. coli strains and a clinical isolate of Stenotrophomonas maltophilia (S. maltophilia), we observed that serum-induced inner membrane disruption correlated with bacterial killing (Fig 1D and E). Both inner membrane disruption and killing by human serum fully relied on the presence of MAC components but not on the peptidoglycan-degrading lysozyme (Figs 1D and E, and EV1C). Taken together, these data suggest that MAC-mediated disruption of the inner membrane is an essential requirement for killing of Gram-negative bacteria in human serum. Purified MAC components lack the bactericidal activity of serum To assess how the MAC can damage both membranes, we used purified MAC components instead of serum. Although nascent C5b is unstable, rapid association with C6 (Cooper & Müller-Eberhard, 1970; Hadders et al, 2012) leads to formation of stable C5b6 complexes that initiate the assembly of the MAC (C5b6-9; Hadders et al, 2012; Serna et al, 2016; Sharp et al, 2016; Bayly-Jones et al, 2017; Fig 2A). Such stable C5b6 complexes can be generated by activating C5 and C6 on activating surfaces (in C7-deficient serum) and subsequently purify released C5b6 from the supernatant (van den Berg, 2000). Upon incubation with C7, C8, and C9, such purified, preassembled C5b6 complexes can form MAC pores in various cell types. This protocol was recently used for structure determination of the MAC following its formation in liposomes (Serna et al, 2016; Menny et al, 2018). In concordance with literature, we also observed that preassembled C5b6 complexes can associate with proteins C7, C8, and C9 to assemble lytic MAC pores (henceforward denoted as C5b6MACs) in liposomes (Fig EV2A; Sharp et al, 2016) and mammalian erythrocytes [human (Fig 2B) and rabbit (Fig EV2B (Lachmann & Thompson, 1970))]. Figure 2. Purified MAC components lack the bactericidal activity of serum Purified MAC (denoted as C5b6MAC) can be formed by mixing preassembled C5b6 complexes with C7, C8, and C9. Lysis of human erythrocytes after exposure to a concentration range of preassembled C5b6 in the presence of 100 nM C7. After washing, erythrocytes were exposed to 20 nM C8 and 100 nM C9 for 30 min after which the OD405 nm of the supernatant was measured. Bacterial viability of three Gram-negative strains after exposure to buffer, 10% human serum or C5b6MAC. Buffer and serum conditions are the same as Fig 1E. Permeabilization of the outer, but not inner membrane of perimCherry/cytoGFP E. coli cells exposed to C5b6MAC (different concentrations of C5b6 with fixed concentrations of C7-C9). Inner membrane damage of three Gram-negative strains exposed to buffer, 10% serum or C5b6MAC. Buffer and serum conditions are the same as Fig 1D. Data information: The cfu/ml (C) and Sytox measurements (E) of “Buffer”, “Serum”, “ΔC5”, “ΔC8”, “Δlysozym”, and “C5b6MAC” were all generated from the same experiment. (B–E) Data represent mean ± SD of 3 independent experiments. (C, E) Statistical analysis was done using a ratio paired two-tailed t-test and displayed only when significant as *P ≤ 0.05, **P ≤ 0.01, ***P ≤ 0.001, or ****P ≤ 0.0001. Normal concentrations of MAC proteins in 100% human serum are ± 375 nM C5, 550 nM C6, 600 nM C7, 350 nM C8, and 900 nM C9. Download figure Download PowerPoint Click here to expand this figure. Figure EV2. MAC assembled from purified C5b6 lacks bactericidal activity Lysis of liposomes after exposure to preassembled C5b6 with or without C7, C8, and C9. Calcein release from liposomes was determined by measuring absorbance at OD340 nm; 0.5% Triton X-100 was used as a positive control. Percentage lysis of rabbit erythrocytes exposed to buffer or C5b6MAC, compared to Milli-Q (MQ) water as control (set at 100% lysis). Killing of E. coli MG1655 after exposure to preassembled C5b6 with or without C7, C8, and C9 (at concentrations similar to (B) or at concentrations exceeding those of 100% serum (highlighted by an arrow); ± 100 nM C5b6, 600 nM C7, 350 nM C8, 900 nM C9). Data information: Data represent mean ± SD of 3 independent experiments. Statistical analysis was done using a ratio paired two-tailed t-test and displayed only when significant as *P ≤ 0.05 or **P ≤ 0.01. Download figure Download PowerPoint However, when preassembled C5b6 triggers MAC formation on bacteria, these pores lack the bactericidal activity of human serum (Fig 2C), even at protein concentrations exceeding those in blood (Fig EV2C). Although C5b6MAC is not bactericidal, it decreased mCherry signals in the entire population, indicating permeabilization of the outer membrane (Fig 2D). While C5b6MAC pores perturb the bacterial outer membrane, they—unlike serum—lack the ability to damage the inner membrane of Gram-negative bacteria (Fig 2D and E). We conclude that C5b6MAC lacks bactericidal activity in spite of its effectiveness in perturbing the outer membrane. Reconstituting bactericidal MAC assembly via surface-bound C5 convertases To identify which additional factors in serum are needed to form bactericidal MAC pores, we next attempted to more closely mimic in vivo MAC assembly via cell-bound C5 convertases (Figs 3A and EV3A; Berends et al, 2014). In serum, antibodies or pattern recognition molecules specifically drive the deposition of C5 convertases onto the target cell surface (Gros et al, 2008; Ricklin et al, 2010; Berends et al, 2014). This occurs in a step-wise manner (Fig EV3A): firstly, all three recognition pathways deposit C3 convertase enzymes that cleave protein C3 into C3b, which covalently attaches to the cell surface via a reactive thioester. At high densities of surface-bound C3b, C3 convertases associate with deposited C3b to form a C5 convertase. Although their surface-specific nature and covalent attachment make it technically challenging to generate C5 convertases in a purified manner, we here show that pre-incubation of bacteria with human serum devoid of C5 (ΔC5 serum) results in functionally active (mainly alternative pathway) C5 convertases on the bacterial surface (Fig EV3B–D). Figure 3. Reconstituting bactericidal MAC assembly via surface-bound C5 convertases Schematic overview for Conv-MAC formation. Bacteria were labeled with C5 convertases by pre-incubation with C5-deficient serum (Fig EV3). Following a washing step (@), convertase-labeled bacteria were incubated with uncleaved C5, C6, C7, C8, and C9 (termed “Conv-MAC”). Bacterial viability of convertase-labeled bacterial strains exposed to buffer (Conv) or C5-C9 (Conv-MAC). Bacterial viability of convertase-labeled E. coli MG1655 exposed to a concentration range of C5 in the presence of 100 nM C6, 100 nM C7, 20 nM C8, and 100 nM C9. “Ctrl” indicates bacteria that are pretreated with heat-inactivated ΔC5 serum. Dotted line represents the detection limit of the assay. Bacterial viability of convertase-labeled E. coli MG1655 exposed to C5-C9 or conditions lacking one MAC component. As an extra control, convertase formation was blocked during ΔC5 serum incubation by adding 5 μM compstatin. Bacterial viability of E. coli MG1655 exposed to FB depleted serum in the presence of 20 μg/ml OmCI (to deposit C4b and C3b without Bb). After washing, bacteria were exposed to C5-C9 in the presence or absence of C1 and C2 (to generate classical pathway C5 convertases, C4b2aC3b). Data information: (B–E) Data represent mean ± SD of 3 independent experiments. (B, D, E) Statistical analysis was done using a ratio paired two-tailed t-test and displayed only when significant as *P ≤ 0.05, **P ≤ 0.01, ***P ≤ 0.001, or ****P ≤ 0.0001. Download figure Download PowerPoint Click here to expand this figure. Figure EV3. Successful labeling of the bacterial surface with C5 convertases A. Schematic overview of complement activation and MAC formation on the bacterial membrane. Different recognition pathways (classical, lectin, and alternative) generate C3 convertases (C4b2a in the classical/lectin pathway, C3bBb in the alternative pathway) on the target cell surface that cleave the major complement protein C3 into C3b. C3b covalently attaches to the cell surface via a reactive thioester. At high C3b densities, C3 convertases associate with deposited C3b to form C5 convertases (C4b2aC3b in the classical/lectin pathway, C3bBbC3b in the alternative pathway). The C5 convertase then catalyzes conversion of C5 into C5a and C5b. C5b triggers MAC formation by sequential binding to C6, C7, C8, and multiple copies of C9. B. Incubation of E. coli MG1655 with a concentration range of C5-depleted serum (ΔC5 serum) results in surface labeling with alternative pathway convertases (C3bBbC3b, evidence by flow cytometry analysis of surface-bound C3b and Bb). C, D. Successful labeling of E. coli with C5 convertases. (C) E. coli MG1655 was pre-incubated with 10% ΔC5 serum (labeled as: C5 convertase), heat-inactivated ΔC5 serum (Ctrl), or ΔC5 serum supplemented with 5 μM compstatin (Ctrl). After washing, C3b deposition was measured by flow cytometry. (D) Bacteria were labeled as described in (C) and (after washing) incubated with a concentration range of C5. Release of C5a into supernatants was measured by a calcium flux-based assay (Bestebroer et al, 2010). Data information: (B–D) Data represent mean ± SD of 3 independent experiments. (B) Statistical analysis was done using a ratio paired two-tailed t-test and displayed only when significant as **P ≤ 0.01 or ***P ≤ 0.001. Download figure Download PowerPoint When such convertase-labeled bacteria were next washed and then incubated with uncleaved C5 and components C6, C7, C8, and C9, we found that the resulting MAC pores (denoted as Conv-MAC) effectively killed bacteria (Fig 3B) in a C5 dose-dependent manner (Fig 3C). As is the case in vivo, C5 convertases here were essential for bacterial killing, since no killing was observed when bacteria were pre-incubated with convertase-negative serum (heat-inactivated ΔC5 serum (Fig 3C), which lacks the capacity to deposit C3b (Fig EV3C) and to convert C5 (Fig EV3D). In addition, bacterial killing was fully inhibited when convertase formation was blocked by compstatin (Sahu et al, 1996; Fig 3D), which specifically inhibits surface deposition of C3b (Fig EV3C) and formation of functional C5 convertases (Fig EV3D). The here observed bacterial killing was MAC-specific, as it required the presence of C5-C9 to be fully effective (Fig 3D). In the presence of C5-C8 alone (no C9), bacterial killing was much less effective but not insignificant (Fig 3D), supporting previous reports on bactericidal effects of C5b-8 in the absence of C9 (O'Hara et al, 2001). Similar to these results based on alternative pathway C5 convertases, specific labeling of bacteria with classical/lectin pathway C5 convertases also led to bacterial cell death upon incubation with C5-C9 (Fig 3E). Altogether, these data demonstrate that purified MAC can kill bacteria when its assembly is driven by cell-bound C5 convertases. MAC assembly via surface-bound C5 convertases leads to inner membrane damage Given that MAC assembly via surface-bound C5 convertases results in bacterial cell death, we assessed whether it also results in inner membrane damage. To study this, perimCherry/cytoGFP bacteria were labeled with C5 convertases of the alternative pathway, washed, and next incubated with components C5-C9, as described above. In concordance with its bactericidal activity, we found that convertase-driven MAC assembly perturbed both the outer and inner membrane of perimCherry/cytoGFP E. coli, in a C5 dose-dependent manner (Fig 4A). In accordance with the killing data in Fig 3C and D, we observed that inhibition of C5 convertase formation via heat inactivation or by adding compstatin prevented MAC-mediated inner membrane damage (Fig 4B). Furthermore, C5 convertase-generated MAC pores (Conv-MAC) also induced inner membrane damage in wild-type E. coli and S. maltophilia strains (Fig 4C). Consistent with the flow cytometry results, confocal microscopy further confirmed that the combination of surface-bound C5 convertases and MAC induces inner membrane damage in bacteria (Fig 4D). In conclusion, when the MAC is assembled from purified C5-C9 by surface-bound convertases, these pores trigger inner membrane damage and subsequent bacterial killing. Figure 4. MAC assembly via surface-bound C5 convertases leads to inner membrane damage Outer membrane damage (mCherry intensity) and inner membrane damage (% Sytox positive) of convertase-labeled perimCherry/cytoGFP E. coli cells incubated with a concentration range of C5 and fixed concentrations of C6-C9. Inner membrane damage of perimCherry/cytoGFP E. coli exposed to a concentration range of ΔC5 serum and, after washing, to C5-C9. As controls, bacteria were incubated with heat-inactivated ΔC5 serum or 5 μM compstatin was added to the ΔC5 serum to block C3b deposition. Inner membrane damage of three different convertase-labeled bacteria exposed to buffer (Conv) or C5-C9 (Conv-MAC). Confocal microscopy images of convertase-labeled perimCherry/cytoGFP E. coli exposed to buffer (Conv) or C5-C9 (Conv-MAC). Unlabeled bacteria exposed to 1% serum served as control. Green = GFP, red = To-pro-3 DNA dye. Scale bars = 3 μm. Data information: (A–C) Data represent mean ± SD of 3 independent experiments. (C) Statistical analysis was done using a ratio paired two-tailed t-test and displayed only when significant as *P ≤ 0.05 or **P ≤ 0.01. Download figure Download PowerPoint Local assembly of C5b6 by surface-bound C5 convertases is required for bacterial killing Having established a protocol to generate bactericidal MACs under semi-purified conditions, we next investigated the difference between the non-bactericidal MACs formed by preassembled C5b6 and fully functional, convertase-generated MACs formed from C5 and C6. To this end, we labeled bacteria with C5 convertases as described above and subsequently generated MACs by incubation with preassembled C5b6 and C7-C9 (Conv-C5b6MAC) or by incubation with uncleaved C5 and C6 and C7-C9 (Conv-MAC; Fig 5A). In this direct comparison, only the Conv-MAC killed bacteria (Fig 5B) and triggered inner membrane damage (Fig 5C), whereas both Conv-C5b6MAC and Conv-MAC triggered outer membrane damage (Fig 5D). These results indicate that the role of C5 convertases extends beyond the cleavage of C5 into C5a and C5b: In particular, the C5 convertase should be present on the cell surface and locally assemble C5b6 to generate bactericidal pores. Interestingly, and fully consistent with the lytic function of C5b6MAC on liposomes (Fig EV2A) and mammalian erythrocytes (Figs 2B and EV2B), local assembly of C5b6 by surface-bound C5 convertases was not essential to kill human cells (Fig EV4; note that this experiment was performed on human (HAP1) cells deficient in complement regulators CD46, CD55, CD59 (Thielen et al, 2018) that, if present, would prevent any C3b or MAC deposition on the ce
更多查看译文
关键词
bacterial killing,membrane attack,complement,c5
AI 理解论文
溯源树
样例
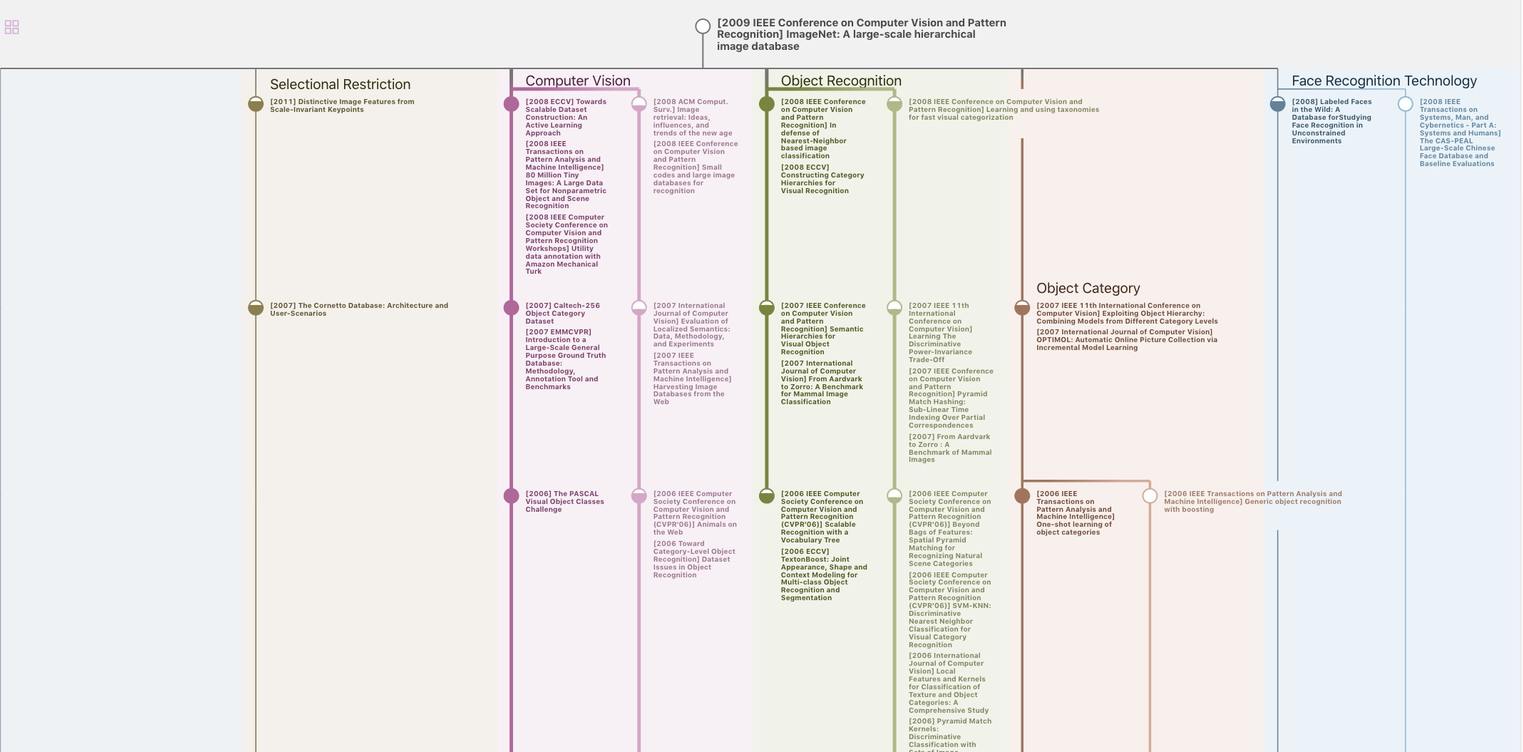
生成溯源树,研究论文发展脉络
Chat Paper
正在生成论文摘要