Post-operative minimal residual disease models to study metastatic relapse in soft-tissue sarcoma patient-derived xenografts.
Clinical and translational medicine(2023)
摘要
Soft-tissue sarcomas (STS) are rare, heterogeneous cancers comprising 1% of adult and 15% of paediatric malignancies. Despite optimal treatment, 50%−80% of patients metastasize, even when they attain a status of minimal residual disease (MRD). MRD is achieved through multimodal treatment involving primary tumour resection with wide negative margins. In metastatic setting, systemic therapies are palliative and response rates are low (15%–20%).1, 2 As a result of these poor outcome data, there is a strong need for translationally relevant patient-derived models. Patient-derived xenografts (PDX) are used to investigate novel therapies and guide personalized treatment response.3 Yet, many PDX models do not reflect the clinical behaviour of human tumours.4 For STS, commonly used PDX still fail to predict the clinical efficacy of (novel) drugs and indeed, MRD status and subsequent metastatic progression have been poorly modelled in STS-PDX5 (Table S1). Moreover, currently available STS-PDX models have not been comparatively assessed. In this study we aimed to address this translational gap by creating PDX that mimic MRD status (MRD-PDX), followed by metastatic relapse and examine the most appropriate model. We first engrafted tumour tissue derived from five high-grade STS patients (Table S2) and resected the subsequent primary tumour at a size of 250-450 mm3 using limb amputation to obtain negative surgical margins,6 similar to the patient's treatment (Figure 1A). The impact of the site of transplantation (orthotopic [O-PDX] vs subcutaneous [SC-PDX]) and immunodeficiency status of the host animal (NOD scid gamma [NSG] vs Swiss nu/nu mice) on primary tumour growth, MRD and disease progression (local recurrence and metastasis) were directly compared for four patients (Figure 1B). PDX were followed up to 1 year after tumour resection. MRI monitored primary tumour growth, MRD and metastatic relapse (Figure 1A). Histopathology and copy number variation (CNV) sequencing evaluated tumour characteristics. Orthotopic tumours had a range of macroscopic and radiologic differences compared to subcutaneous STS, including irregular shape, heterogeneity, lack of circumscription, increased vascularization and unclear margins with the surrounding tissue (Figure 2A). Histologically, orthotopic tumours were uncapsulated, showed invasion of the surrounding tissue, vascular and neural encasement and necrosis. Alpha smooth muscle actin (αSMA), which can be indicative for cancer-associated fibroblasts,7 was positive homogenously throughout orthotopic tumours, and only peripherally in subcutaneous tumours derived from the same patient (Figure 2B). Overall engraftment ratio for all models was 61.1% (25%–91.7%) and was highest in O-NSG (Figure 2C). Probability of tumour growth (Kaplan–Meier method) showed no significant difference in engraftment efficiency between SC- and O-PDX (p = .156, Figure 2D), but was significantly more efficient in NSG, compared to Swiss nu/nu (p = .0025, Figure 2E). Overall disease progression ratio for all models was 27.8% (0%–50%) and was again highest in O-NSG (Figure 2F). Probability of disease progression was significantly higher in O-PDX, compared to SC-PDX (p = .006, Figure 2G), but was not significantly impacted by mouse strain (p = .382, Figure 2H). PDX tumour volume at time of resection was investigated as confounding factor for disease progression but was not significantly different between PDX that did and did not metastasize (p = .351). Resection margins were negative in all animals. The O-NSG model was selected as the most applicable and usable as MRD-PDX due to the high probability of tumour take and disease progression, with an overall MRD period of 14.61 ± 6.09 weeks (Figure 3). In contrast, only 1 out of 16 SC-PDX showed disease progression (local recurrence). This model was derived from a patient lung metastasis (EOS/045/M) and had an MRD period of 51.4 weeks. Although disease progression in O-Swiss did not significantly differ from O-NSG, we do not support this as MRD-PDX model. First, the take ratio is lower, which reduces the usability. Second, out of the three O-Swiss that showed metastases, only one had been preceded by a primary tumour (MRD period of 36.5 weeks). The two others developed metastases without clinically and radiologically detectable primary tumour growth, which was never the case in O-NSG. Our observations add to the evidence supporting that the microenvironment at the implantation site determines tumour behaviour.4 Based on these results, we developed second-generation O-NSG by implanting either primary tumour (G2 O-NSG) or metastasis tissue (G2 mO-NSG) derived from first-generation (G1) O-NSG (Figure 1C). Take ratio was 100% for both G2 and tumour growth was significantly faster compared to G1 (Figure 4A), as observed in other tumours.8 G2 mO-NSG exhibited a more aggressive phenotype with faster-developing metastases (MRD period of 2.6 ± .084 weeks) (Figure 4B). Similar metastatic patterns were observed in G1, G2 mO-NSG and patients (Table S3, Supporting Information). The histological phenotype of STS (atypical, pleomorphic spindle cells with abundant mitotic figures) and immunohistochemistry pattern were maintained throughout the different PDX passages, including PDX metastases (Figure 4C). Remarkably, histologic architecture was highly similar between patient and PDX metastases. The genome difference, characterized by CNV, was only 0%–3.4% between G1 and G2 O-NSG primary tumours, suggesting that epigenetic or transcriptional adaptations drive the higher efficiency rates of the next generations. As expected from previous reports,9 discrete changes in CNV were observed between the patient's primary tumour sample and PDX passages (genome difference 23.58%–28.43%) (Figure 4D). Potential applications of MRD-PDX include investigating the impact of surgery on disease progression, biomarker studies to determine the detection limit of MRD; evaluating therapies maintaining MRD-status and modelling metastasis-targeted therapies.4 Unfortunately, due to the absence of an intact immune system, evaluation of anti-PD1 therapy, which looks promising for some STS subtypes,10 is not possible. Although primary tumour-derived MRD-PDX developed metastasis faster than the corresponding patients in this study, MRD-PDX require a long observation time, and as it remains impossible to predict which high-grade patients are at risk for metastasis, this hampers the usability in personalized medicine. Due to the heterogeneity of STS, a one-size-for-all solution cannot be expected, and we strongly believe that these models are a valuable, but complementary addition to other available preclinical models. Further validation in larger STS MRD-PDX cohorts will be an important contribution to the current available data. In conclusion, MRD-PDX developed by orthotopic implantation in NSG mice followed by surgical removal of the primary tumour accurately simulate the clinical behaviour of STS. This model holds MRD-specific benefits and should not be neglected when evaluating novel therapies. The authors would like to thank the nurses working at the operating theatre of the Ghent University Hospital and the residents of the pathology department for assisting in the collection of tumour material. Moreover, the authors would like to thank Goedele Ronse for aiding with the recruitment of patients. The authors especially want to thank all patients who consented to participate in this study. The authors declare no conflicts of interest. Please note: The publisher is not responsible for the content or functionality of any supporting information supplied by the authors. Any queries (other than missing content) should be directed to the corresponding author for the article.
更多查看译文
关键词
metastatic relapse,sarcoma,xenografts
AI 理解论文
溯源树
样例
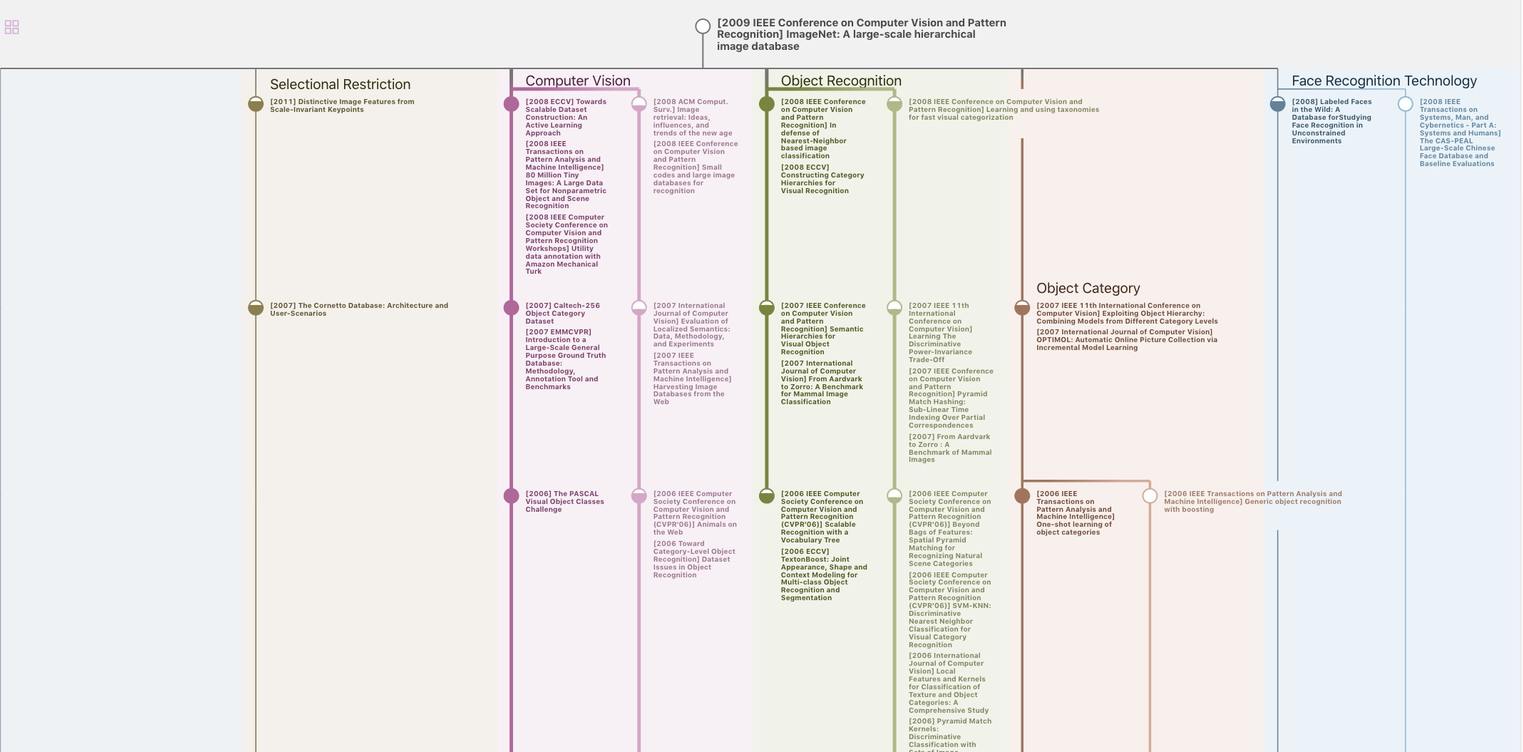
生成溯源树,研究论文发展脉络
Chat Paper
正在生成论文摘要