2H-MoS 2 Modified Nitrogen-Doped Hollow Mesoporous Carbon Spheres as the Efficient Catalytic Cathode Catalyst for Aprotic Lithium-Oxygen Batteries
Renewables(2023)
摘要
Open AccessRenewablesRESEARCH ARTICLES20 Jan 20232H-MoS2 Modified Nitrogen-Doped Hollow Mesoporous Carbon Spheres as the Efficient Catalytic Cathode Catalyst for Aprotic Lithium-Oxygen Batteries Zhaorui Zhou, Lanling Zhao, Yao Liu, Deyuan Li, Qing Xia, Jun Wang, Zidong Zhang, Xue Han, Yuxin Long, Yiming Zhang, Yebing Li and Shulei Chou Zhaorui Zhou Key Laboratory for Liquid-Solid Structural Evolution and Processing of Materials (Ministry of Education), Shandong University, Jinan 250061 Google Scholar More articles by this author , Lanling Zhao School of Physics, Shandong University, Jinan 250100 Google Scholar More articles by this author , Yao Liu *Corresponding authors: E-mail Address: [email protected] E-mail Address: [email protected] E-mail Address: [email protected] Key Laboratory for Liquid-Solid Structural Evolution and Processing of Materials (Ministry of Education), Shandong University, Jinan 250061 Google Scholar More articles by this author , Deyuan Li Key Laboratory for Liquid-Solid Structural Evolution and Processing of Materials (Ministry of Education), Shandong University, Jinan 250061 Google Scholar More articles by this author , Qing Xia Key Laboratory for Liquid-Solid Structural Evolution and Processing of Materials (Ministry of Education), Shandong University, Jinan 250061 Google Scholar More articles by this author , Jun Wang *Corresponding authors: E-mail Address: [email protected] E-mail Address: [email protected] E-mail Address: [email protected] Key Laboratory for Liquid-Solid Structural Evolution and Processing of Materials (Ministry of Education), Shandong University, Jinan 250061 Google Scholar More articles by this author , Zidong Zhang Key Laboratory for Liquid-Solid Structural Evolution and Processing of Materials (Ministry of Education), Shandong University, Jinan 250061 Google Scholar More articles by this author , Xue Han Key Laboratory for Liquid-Solid Structural Evolution and Processing of Materials (Ministry of Education), Shandong University, Jinan 250061 Google Scholar More articles by this author , Yuxin Long Key Laboratory for Liquid-Solid Structural Evolution and Processing of Materials (Ministry of Education), Shandong University, Jinan 250061 Google Scholar More articles by this author , Yiming Zhang Key Laboratory for Liquid-Solid Structural Evolution and Processing of Materials (Ministry of Education), Shandong University, Jinan 250061 Google Scholar More articles by this author , Yebing Li Key Laboratory for Liquid-Solid Structural Evolution and Processing of Materials (Ministry of Education), Shandong University, Jinan 250061 Google Scholar More articles by this author and Shulei Chou *Corresponding authors: E-mail Address: [email protected] E-mail Address: [email protected] E-mail Address: [email protected] Institute for Carbon Neutralization, College of Chemistry and Materials Engineering, Wenzhou University, Wenzhou 325035 Google Scholar More articles by this author https://doi.org/10.31635/renewables.022.202200004 SectionsSupplemental MaterialAboutAbstractPDF ToolsAdd to favoritesDownload CitationsTrack Citations ShareFacebookTwitterLinked InEmail Developing excellent cathode catalysts with superior catalytic activities is essential for the practical application of aprotic lithium-oxygen batteries (LOBs). Herein, we successfully synthesized nitrogen-doped hollow mesoporous carbon spheres encapsulated with molybdenum disulfide (MoS2) nanosheets as the cathode catalyst for rechargeable LOBs, and the relationship between the battery performance and structural characteristics was intensively researched. We found that the synergistic effect of the nitrogen-doped mesoporous carbon and MoS2 nanosheets endows superior electrocatalytic activities to the composite catalyst. On the one hand, the nitrogen-doped mesoporous carbon could enable fast charge transfer and effectively accommodate more discharging products in the composite skeleton. On the other hand, the thin MoS2 nanosheets could promote mass transportation to facilitate the revisable formation and decomposition of the Li2O2 during oxygen reduction reaction and oxygen evolution reaction, and the side reactions were also prevented, apparently due to their full coverage on the composite surfaces. As a result, the catalytic cathode loaded with 2H-MoS2-modified nitrogen-doped hollow mesoporous carbon spheres exhibited excellent electrochemical performance in terms of large discharge-/charge-specific capacities with low overpotentials and extended cycling life, and they hold great promise for acting as the cathode catalyst for high-performance LOBs. Download figure Download PowerPoint Introduction Overuse of fossil fuel and the resulting environmental pollution have been urgent issues for governments around the world in recent decades, and scientists and researchers around the world have focused considerable attention on renewable and sustainable energy sources to mitigate the pressure from these issues,1–4 studying such areas as solar energy, tidal energy, wind energy, and so on.5–8 With the research developing, finding an effective system that can convert and store the energy is the key to realizing the feasible and effective utilization of environmentally protective solutions. Currently, lithium-ion batteries (LIBs) are employed as the most successful energy storage equipment, which are widely used in mobile phones, laptops, electric vehicles, and so on.9–13 However, the future practical applications of LIBs are severely constrained by their limited energy density.14–16 Since aprotic lithium-oxygen batteries (LOBs) were first proposed in 1996,17 they have attracted considrable attention owing to their high theoretical energy density of 3623 Wh kg−1, which is 10 times higher than that of LIBs and close to their gasoline counterpart.18–20 However, there are still many problems that inhibit the practical applications of rechargeable LOBs, mainly involving limited rate capability, poor cycling stability, and low practical energy density.21,22 Researchers have done diverse studies to solve the above problems, including construction of efficient catalytic cathodes,23–25 exploitation of safe and stable electrolytes,26–28 and modification of the Li anode.29–31 Among these solutions, the bi-functional catalytic cathode is believed to be key to enhancing LOB performance by accelerating the sluggish kinetics of oxygen reduction reaction (ORR) and oxygen evolution reaction (OER).32–34 Up until now, the electrochemical catalytic activities of many materials have been studied as cathodes in LOBs, including carbons,35–38 precious metals,39 transition metal sulfides,40,41 transition metal oxides,23,42 metal phosphides,43 and perovskites.44–46 It is evident that molybdenum disulfide (MoS2) is a potential candidate as the cathode catalyst for LOBs due to its low cost, high stability, tunable electron structure, and excellent electrocatalytic activities.47–49 In recent years, plenty of MoS2-based materials have been synthesized successfully through diverse structural engineering strategies as the catalytic materials for LOBs, including precious metals modified MoS2,50 amorphous MoS2,49 MoS2-coated carbon composites,51,52 phase modulated MoS2,48,53 and defected MoS2.41 As we all know, three different phases of trigonal prismatic 2H, octahedral 1T, and distorted octahedral 3R are normally found in MoS2, and their electrochemical activities are closely associated with the phase components.41,53,54 Notably, 2H-MoS2 is not only the most stable phase in room temperature, but it also features superior electrochemical catalytic activities compared with those of the other two phases.48 However, the electrical conductivity of 2H-MoS2 is poor due to its semiconductive properties, which largely limits its electrocatalytic activities and hinders practical application in LOBs. To solve this problem, combining 2H-MoS2 with conductive carbon materials was proposed to enhance the electrical conductivity and promote their overall electrocatalytic properties for Li-O2 catalysis. Li et al.41 synthesized a core-shell MoS2-x@CNTs composite via sulfur defect engineering and obtained a cycle life of 666 at 1000 mA g−1 with a limited specific capacity of 500 mAh g−1 in LOBs. Long et al.51 developed a three-dimensional flower-like MoS2@carbon nanotube via a hydrothermal treatment-assisted method, which cycled 141 rounds at a current density of 500 mA g−1 with a limited capacity of 1000 mAh g−1. Liu et al.52 fabricated a three-dimensional carbon-supported MoS2 with sulfur defects and achieved a cycle life of 123 rounds at 500 mA g−1 with a limited specific capacity of 1000 mAh g−1. Evidently, the construction of unique architecture with abundant free room and rich pores throughout carbon materials is tunable,55 and this contributes to advancing mass transport and mitigating the striking volume changes resulting from the formation and accumulation of discharge products during the cycling of LOBs.35,41 In addition, we found that the presence of abundant mesopores on the carbon matrix can also facilitate the penetration of electrolytes on the cathode surfaces, accommodate more discharging products, and promote mass transfer, resulting in accelerated reaction kinetics.35,56 Moreover, we found that the introduction of nitrogen on carbon materials not only improved the electrical conductivity, but it also largely increased the active sites on their surfaces.57 Furthermore, the excellent wettability of the carbon matrix induced by doping nitrogen enabled their uniform and tight contact with the reactants in the synthesis process,58 and this is favorable for realizing the full coverage of the in situ growth of materials on their surfaces, effectively inhibiting the side reactions involved with carbon materials.59,60 Herein, 2H-MoS2 anchored on nitrogen-doped hollow mesoporous carbon spheres were synthesized via the hydrothermal and annealing method. There are three advantages expected with this composite as an active cathode material for LOBs. First, the 2H-MoS2 with thin-layered nanosheet provided abundant catalytic active sites toward ORR and OER to accelerate the sluggish reaction kinetics. Second, the nitrogen-doped mesoporous carbon matrix not only enhanced the electrical conductivity of the catalyst, but it also offered enough space for storing discharging products, which remarkably enlarged the specific capacities and enhanced the cycling stability of the LOBs. Finally, nitrogen-doped hollow mesoporous carbon spheres were fully coated with MoS2 nanosheets, effectively preventing the contact of carbon matrix with the electrolytes and discharge products to effectively inhibit the formation of by-products and improve the cycling performance of LOBs during discharging and charging processes. Results and Discussion The overall synthetic process of nitrogen-doped mesoporous carbon-coated MoS2 ([email protected]2) is shown in Scheme 1. Clearly, nitrogen-doped mesoporous polymer (NMP) was synthesized through an emulsion-induced interface anisotropic assembly method. Then, the MoS2 nanosheets were successfully grown in situ on the surfaces of NMP by a hydrothermal method to obtain [email protected]2. Successively, it was annealed in an Ar atmosphere at 750 °C for 2 h to carbonize the polymer matrix and enhance the crystallinity of MoS2. As-prepared products during the synthetic process were recorded by digital photos (see Supporting Information Figure S1). Scheme 1 | Schematic illustration for the synthetic process of [email protected]2. Download figure Download PowerPoint The morphologies and structures of different samples were characterized by field emission scanning electron microscopy (FESEM) and transmission electron microscopy (TEM) techniques. The FESEM image of the NMP precursor displays a cheese jar morphology with a uniform particle size of ∼500 nm (Figure 1a). The Fourier transform infrared (FT-IR) spectrometer spectrum of NMP (see Supporting Information Figure S5) exhibits characteristic peaks of NMC with typical functional groups, including N–H, –OH, C–O, and C–N that were quite consistent with the data previously reported.61 After being annealed, NMP transformed into NMC with a little bit of size expansion (see Supporting Information Figure S2a), where uniform pores with the diameter of ∼10 nm can be clearly observed (see Supporting Information Figure S4). The FESEM image of pure MoS2 was also included as a comparison (see Supporting Information Figure S2b). In addition, we employed commercial MoS2 nanoflakes as a reference material to compare with the other samples in this work, and their X-ray diffraction (XRD) pattern and FESEM image are provided in Supporting Information Figure S3. FESEM and TEM images of [email protected]2 show that the thin MoS2 nanosheets were successfully anchored on the surfaces of NMC (Figure 1b,c). The high-resolution TEM (HRTEM) image (Figure 1d) with a corresponding lattice distance profile (Figure 1e) indicates that the interlayer spacing of MoS2 is 0.64 nm, which corresponds to the (002) planes of MoS2,51,62 and that of the carbon matrix is 0.34 nm, which is indexed to the (002) planes of the graphitic carbon.10,41 In addition, the selected area electron diffraction (SAED) pattern (Figure 1f) demonstrates the existence of (002), (101), (103), and (008) planes, corresponding to the reference patterns of MoS2 (JCPDS No. 87-2416). To investigate the element distribution on [email protected]2, element distribution mapping images were obtained (Figure 1g), showing that the Mo, S, C, N, and O elements were uniformly distributed on the surfaces of [email protected]2. Figure 1 | (a) FESEM image of NMP precursor, (b) FESEM image, and (c) TEM image. (d) HRTEM image with (e) lattice distance profile, (f) SAED result, and (g) EDX mapping data of [email protected]2. Download figure Download PowerPoint The XRD results (Figure 2a) illustrate that all peaks of [email protected]2 locate at the same position as those of pure MoS2, which match well with reference peaks of MoS2 (JCPDS No.87-2416). For [email protected]2, the peak at 14.3° exhibits a slight shift to a lower degree compared with that of the pure MoS2, indicating an expansion in the MoS2 (002) lattice spacing, caused most likely by the defects in [email protected]2.41,52 Raman spectra of [email protected]2, pure MoS2, and NMC are shown in Figure 2b. Specifically, two wide peaks can be traced at around 1360 and 1600 cm−1, respectively, corresponding to D and G bands of carbon materials. As we all know, the D band is associated with the disorder and defects in the graphitized carbon while the G band represents the in-plane stretching vibration of sp2-bonded hybridization of carbon atoms, with the intensity ratio of the D band and G band indicating the extent of disorder in the carbon structure.35 As reported in previous research, higher ID/IG normally originates from the graphitic carbon undergoing a reduction reaction, forming abundant defects and disordered structures.63,64 In our synthetic method, the [email protected]2 was synthesized via a hydrothermal method using sodium molybdate and thiourea as the reactants (4Na2MoO4 + 15CS(NH2)2 + 6H2O → 4MoS2 + Na2SO4 + 6NaSCN + 24NH3 + 9CO2). The reducibility of thiourea and ammonia gas promoted the reduction reaction and generated more defects and disordered structures, resulting in a higher ID/IG after coating MoS2. Furthermore, the ID/IG of [email protected]2 and NMC was 1.063 and 0.971, respectively, which mainly stems from the MoS2 nanosheet coating on the carbon surfaces. It is apparent that the peaks around 380 and 408 cm−1 appear in the Raman data of both [email protected]2 and pure MoS2, which are assigned to the in-plane vibrational mode (E12g) and out-of-plane vibrational mode (A1g) of 2H-MoS2. It was reported that the energy differences between them can be used to roughly detect the layers of MoS2 nanosheets, and the relationship between the energy differences and the number of MoS2 layers was positively correlated.65,66 As shown in the inset, the energy difference of [email protected]2 is 24.3 cm−1, which is smaller than the 27.5 cm−1 of pure MoS2. This demonstrates that the MoS2 nanosheets on [email protected]2 was thinner than those of pure MoS2. Moreover, the intensity ratio of E12g/A1g normally reflects the exposure degree of MoS2. Namely, the smaller the ratios, the more the edge sites are exposed.41 As shown in Figure 2b, the E12g/A1g ratio of [email protected]2 was 0.3275, lower than the value of pure MoS2, implying that the introduction of nitrogen into the carbon matrix can avoid the stacking of MoS2 nanosheets with highly homogenous distribution on NMC surfaces. Figure 2 | (a) XRD patterns, (b) Raman spectra of different samples, (c) TGA result, and (d)–(h) XPS data of [email protected]2. Download figure Download PowerPoint Thermogravimetric analysis (TGA) measurement was carried out to determine the content of MoS2 in [email protected]2 (Figure 2c). As shown in Figure 2c, 31.4% mass loss between 370 and 600 °C was detected, caused by combustion of NMC and oxidation of MoS2, and the final residuum is believed to be MoO3.67 It thus can be calculated that the precise content of MoS2 in the composite is 76.28%. The N2 adsorption/desorption isotherms (see Supporting Information Figure S7) indicate that the [email protected]2 displayed a typical-IV isotherm curve with the specific area of 34.676 m2 g−1, providing a sample of a mesoporous structure with the pore size distribution centered at 3.8 nm. This architecture allowed sufficient active sites to be exposed, which promoted the formation and decomposition of discharging products and allowed for more space to accelerate mass transfer and accommodate more discharging products. Much more than that, the abundant mesopores promoted the penetration of the electrolyte more effectively, which remarkably boosted electrocatalytic reactions. For comparison, the specific areas of NMC and pure MoS2 were also shown to be 17.528 and 26.497 m2 g−1, respectively. These discrepancies in porosity and specific surface area can be attributed to the morphology. Compared with the sphere particles composed of heavy MoS2 flakes and a dense structure of NMC, the [email protected]2 showed a hollow structure coated with thick flakes, resulting in a higher specific surface area. X-ray photoelectron spectroscopy (XPS) testing was carried out to analyze the surface chemical composition and electron state of [email protected]2. Its XPS survey spectrum (Figure 2d) confirmed that there were no additional elements existing but only Mo, S, C, and O elements. The high-resolution XPS spectrum of Mo 3d orbit (Figure 2e) exhibits that two peaks at around 232.75 and 229.55 eV are assigned as 3d3/2 and 3d5/2 orbitals, which indicate the existence of Mo4+.68 The peak at about 236 eV is ascribed as Mo6+, which arose from the oxidation of a few MoS2 compounds on the surface of composites. The peak at around 226.75 eV belongs to S 2s orbitals, demonstrating the Mo–S bonds in the composite.61 For comparison, the high-resolution XPS spectrum of pure MoS2 shows a positive shift about 0.3 eV in Mo 3d orbitals (see Supporting Information Figure S6), which mainly came from the defects introduced by ion irradiation and significantly altered the band structure.69,70 This result is consistent with the Raman data, in which one shoulder appeared as the left E 2 g 1 mode and another emerged as the right A1g mode to be assigned as defect signals.71 For the high-resolution XPS S 2p spectrum (Figure 2f), the double peaks at around 163.65 and 162.45 eV can be indexed to the S 2p1/2 and S2p3/2 orbitals of S2−, respectively.72,73 The high-resolution XPS spectrum of C 1s exhibits three peaks at 287.25, 286.3, and 284.8 eV, which are attributed to the C–O, C–N, and C–C bonds on NMC, respectively (Figure 2g).74–76 Likewise, three obvious peaks at around 400.95, 398.6, and 397.3 eV are owing to the graphitic N, pyridinic N, and pyrrolic N, respectively, illustrating the successful N doping on carbon matrix (Figure 2h).77 Different samples were assembled in LOBs as the cathode catalysts to investigate their electrochemical catalytic performance. The galvanostatically initial discharge/charge curves at different current densities within 2.35–4.5 V of [email protected]2 cathodes are shown to investigate the rate capacity performance (Figure 3a). With the increase of current densities, polarization became more distinct, and specific capacities decreased with higher overpotentials. Namely, the [email protected]2 cathodes exhibit specific discharge/charge capacities of 15537.7/15283.6, 14334.3/14155.9, 10138.4/8845.5, and 8441.4/7549 mAh g−1 at current densities of 100, 200, 500, and 1000 mA g−1, respectively. Figure 3 | (a) Rate capability of [email protected]2 cathodes and (b) comparison with other different cathodes. (c) CV curves of different cathodes. (d) Initial specific capacities of different cathodes at 100 mA g−1. (e) Cycling performance of different cathodes at a current density of 100 mA g−1 with a specific capacity of 1000 mAh g−1. Download figure Download PowerPoint Rate capability results (Figure 3b) show the [email protected]2 cathodes display much weaker terminal voltage fluctuations at different current densities ranging from 100 to 1600 mA g−1, compared with those of other cathodes, which confirm its superior rate capability in LOBs. The electrical conductivity of the [email protected]2 composite was measured as 0.74 S cm−1 using four-point probe techniques, which is much higher than that of the pure MoS2 (∼10−4 S cm−1) and approaches to that of NMC (3.25 S cm−1). With the construction of the architecture of the MoS2-wrapped carbon matrix, the electrical conductivity of the [email protected]2 cathode dramatically increased, enabling fast charge transfer and contributing to an improvement in the electrochemical performance. Cyclic voltammetry (CV) curves of different cathodes are displayed in Figure 3c. The cathodic peaks at around 2.25–2.5 V are related to the formation of Li2O2 during ORR, and anodic peaks at about 3.5–4.5 V are associated with the decomposition of Li2O2 during OER. Among those cathodes, the [email protected]2 cathode delivered much higher anodic/cathodic currents and larger areas of curve integration, demonstrating that the [email protected]2 can effectively accelerate the composition and decomposition of Li2O2 during cycling. There are two peaks located at 3.5 and 4.3 V detected in the profiles of [email protected]2 cathode in the anodic scan, corresponding to two platforms of galvanostatic charging curve in Figure 1a, which are associated with the delithiation of Li2O2 crystalline (eq 1) and decomposition of Li2-xO2 (eq 2), respectively.78,79 Li 2 O 2 → Li 2 - x O 2 + xLi + + xe − (1) Li 2 - x O 2 → ( 2 - x ) Li + + ( 2 - x ) e − (2) Galvanostatically initial discharge/charge curves of various cathodes at 100 mA g−1 are provided in Figure 3d. It is obvious that the [email protected]2 cathode shows the largest specific capacities and lowest overpotentials, compared with those of other cathodes. In addition to this, we investigated the cycling stability of different cathodes at 100 mA g−1 with a limited capacity of 1000 mAh g−1 (Figure 3e). For commercial MoS2 nanoflakes, their electrocatalytic activity is similar to the pure MoS2 counterpart we synthesized, evidenced by CV results. Its specific capacities are quite limited, mainly due to the dense morphology, also resulting in poor cycling stability. It is worth noting that the electrocatalytic performance of [email protected]2 cathode decayed after 145 cycles while the Ketjen black (KB), NMC, and MoS2 cathodes only present cycle life of 28, 38, and 65 cycles, respectively. The selected galvanostatic discharge/charge curves of different cathodes are depicted in Supporting Information Figure S8, and the [email protected]2 cathode obviously exhibits the superior cycling stability with the lowest overpotentials. The comparison of the electrochemical performance based on MoS2 or carbon-containing cathodes for LOBs is given in Supporting Information Table S1. To investigate the electrochemical reactions of [email protected]2 cathodes that occurred in discharging and charging processes, ex situ XRD, electrochemical impedance spectroscopy (EIS), and FESEM measurements were carried out to study their state evolutions at different stages. The XRD pattern of pristine cathode (Figure 4a) only shows one obvious peak around 54°, which is related to carbon paper. After discharging at 100 mA g−1 with a limited capacity of 1000 mAh g−1, two peaks at around 32.5° and 34.5° appeared, indicating the formation of Li2O2 as discharging products. The XRD pattern after charging almost reverted to that at the pristine state, demonstrating that the [email protected]2 cathode effectively promoted the decomposition of discharging products. In addition to this, there are no other peaks in the XRD spectrum after 130th recharging, which indicates the excellent cycling stability of [email protected]2 cathode. Otherwise, EIS plots in Figure 4b verify the favorable cycling reversibility of the [email protected]2 cathode. The charge-transfer resistance of pristine [email protected]2 cathode is only 56 Ω, and it increased to 150 Ω suddenly after first discharging, which was mainly caused by the formation of discharging products with poor electrical conductivity. As expected, the charge-transfer resistance reverted back to 81 Ω after first recharging, and no obvious changes were found after the 130th recharging, further proving its satisfactory electrocatalytic ability for LOBs. Due to the fast charge transfer and great adsorption strengthening of Li2O2 on [email protected]2 cathode surfaces, the discharged products accumulated on the cathode surfaces and formed a film-like structure. The discharged products membraned the [email protected]2 cathode after discharging at a current density of 100 mA g−1 with a limited capacity of 1000 mAh g−1 (see Supporting Information Figure S9a). After fully recharging (see Supporting Information Figure S9b), the membranous discharged products were decomposed, and the morphology of the [email protected]2 was almost unchanged compared with the original state. Based on the above results, we can conclude that the discharged products can easily be formed and fully decomposed, releasing oxygen and Li+ from the [email protected]2 cathode surfaces. It is also worth noting that the fully covered MoS2 nanosheets effectively prohibited the formation of by-products. Figure 4 | (a) XRD patterns and (b) EIS plots of [email protected]2 cathodes at different states; (c) free diagrams for the ORR/OER and (e) charge density differences of [email protected]2 absorbed different reaction products; (d) total and partial DOS for [email protected]2. Download figure Download PowerPoint Density functional theory (DFT) calculations were conducted to further investigate the adsorption processes and the variation of the electronic structure of different samples. The differential charge density distribution (see Supporting Information Figure S11) on [email protected]2 indicates that the introduction of nitrogen-doped carbon made the Mo layer tend to lose part of its charge, assuming a positive valence state, while N gathered charge, resulting in a negative valence state. Yellow and blue contours in the electron density difference represented the increased and decreased electron density, respectively. The isosurface level was set to be 0.0005 e Bohr−3. As shown in Supporting Information Figure S11a, the formation of delocalized electrons between the inner layer S and the nitrogen-doped area not only accelerated the charge transfer transport in layers, but it also alleviated the anisotropy of electronic structure. Moreover, the calculated Bader charges (see Supporting Information Figure S12) show that the interfaces of the MoS2 and nitrogen-doped carbon induced the charge decrease of Mo and C and the charge increase of S and N, indicating that the charge transferred from the MoS2 layer to the N–C layer. These results satisfactorily explain the peak shift in the high-resolution XPS spectra from the atomic scale. The density of states (DOS) was calculated to further investigate the electronic structure of the samples. According to the previous research,41 the pure 2H-MoS2 exhibits a band gap of 0.59 eV, which indicates its poor metal electrical conductivity. While the projected and total DOS diagram (Figure 4d) demonstrates that [email protected]2 displays a
更多查看译文
关键词
efficient catalytic cathode catalyst,h-mos,nitrogen-doped,lithium-oxygen
AI 理解论文
溯源树
样例
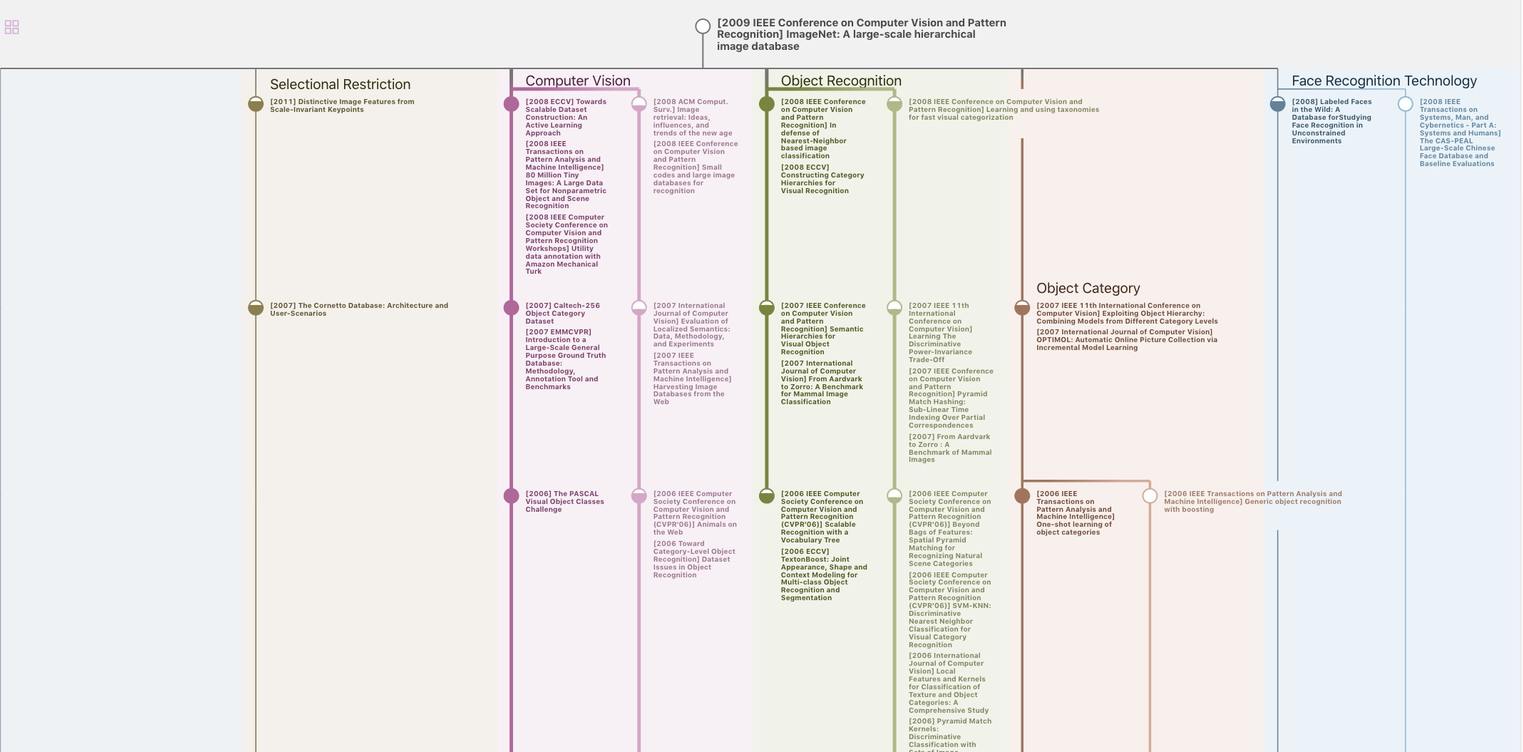
生成溯源树,研究论文发展脉络
Chat Paper
正在生成论文摘要