Aerial Roots of the Leafless Epiphytic Orchid Taeniophyllum Are Specialized for Performing Crassulacean Acid Metabolism Photosynthesis
New phytologist(2023)
Abstract
New PhytologistVolume 238, Issue 3 p. 932-937 LettersFree Access Aerial roots of the leafless epiphytic orchid Taeniophyllum are specialized for performing crassulacean acid metabolism photosynthesis Kenji Suetsugu, Corresponding Author Kenji Suetsugu [email protected] orcid.org/0000-0002-7943-4164 Department of Biology, Graduate School of Science, Kobe University, 1-1 Rokkodai, Nada-ku, Kobe, Hyogo, 657-8501 Japan The Institute for Advanced Research, Kobe University, 1-1 Rokkodai, Nada-ku, Kobe, Hyogo, 657-8501 JapanAuthors for correspondence: emails [email protected] (KS); [email protected] (KT); [email protected] (KK)Search for more papers by this authorRyohei Sugita, Ryohei Sugita Graduate School of Agricultural and Life Sciences, The University of Tokyo, 1-1-1, Yayoi, Bunkyo-ku, Tokyo, 113-8657 Japan Radioisotope Research Center, Nagoya University, Furo-cho, Chikusa-ku, Nagoya, Aichi, 464-8602 JapanSearch for more papers by this authorAkiko Yoshihara, Akiko Yoshihara Department of Biology, Graduate School of Science, Osaka Metropolitan University, 1-1 Gakuen-cho, Naka-ku, Sakai, Osaka, 599-8531 JapanSearch for more papers by this authorHidehito Okada, Hidehito Okada Department of Biology, Graduate School of Science, Kobe University, 1-1 Rokkodai, Nada-ku, Kobe, Hyogo, 657-8501 JapanSearch for more papers by this authorKae Akita, Kae Akita Department of Chemical and Biological Sciences, Faculty of Science, Japan Women's University, Bunkyo-ku, Tokyo, 112-8681 JapanSearch for more papers by this authorNoriko Nagata, Noriko Nagata Department of Chemical and Biological Sciences, Faculty of Science, Japan Women's University, Bunkyo-ku, Tokyo, 112-8681 JapanSearch for more papers by this authorKeitaro Tanoi, Corresponding Author Keitaro Tanoi [email protected] orcid.org/0000-0001-7545-2771 Graduate School of Agricultural and Life Sciences, The University of Tokyo, 1-1-1, Yayoi, Bunkyo-ku, Tokyo, 113-8657 JapanAuthors for correspondence: emails [email protected] (KS); [email protected] (KT); [email protected] (KK)Search for more papers by this authorKoichi Kobayashi, Corresponding Author Koichi Kobayashi [email protected] orcid.org/0000-0003-3726-4790 Department of Biology, Graduate School of Science, Osaka Metropolitan University, 1-1 Gakuen-cho, Naka-ku, Sakai, Osaka, 599-8531 JapanAuthors for correspondence: emails [email protected] (KS); [email protected] (KT); [email protected] (KK)Search for more papers by this author Kenji Suetsugu, Corresponding Author Kenji Suetsugu [email protected] orcid.org/0000-0002-7943-4164 Department of Biology, Graduate School of Science, Kobe University, 1-1 Rokkodai, Nada-ku, Kobe, Hyogo, 657-8501 Japan The Institute for Advanced Research, Kobe University, 1-1 Rokkodai, Nada-ku, Kobe, Hyogo, 657-8501 JapanAuthors for correspondence: emails [email protected] (KS); [email protected] (KT); [email protected] (KK)Search for more papers by this authorRyohei Sugita, Ryohei Sugita Graduate School of Agricultural and Life Sciences, The University of Tokyo, 1-1-1, Yayoi, Bunkyo-ku, Tokyo, 113-8657 Japan Radioisotope Research Center, Nagoya University, Furo-cho, Chikusa-ku, Nagoya, Aichi, 464-8602 JapanSearch for more papers by this authorAkiko Yoshihara, Akiko Yoshihara Department of Biology, Graduate School of Science, Osaka Metropolitan University, 1-1 Gakuen-cho, Naka-ku, Sakai, Osaka, 599-8531 JapanSearch for more papers by this authorHidehito Okada, Hidehito Okada Department of Biology, Graduate School of Science, Kobe University, 1-1 Rokkodai, Nada-ku, Kobe, Hyogo, 657-8501 JapanSearch for more papers by this authorKae Akita, Kae Akita Department of Chemical and Biological Sciences, Faculty of Science, Japan Women's University, Bunkyo-ku, Tokyo, 112-8681 JapanSearch for more papers by this authorNoriko Nagata, Noriko Nagata Department of Chemical and Biological Sciences, Faculty of Science, Japan Women's University, Bunkyo-ku, Tokyo, 112-8681 JapanSearch for more papers by this authorKeitaro Tanoi, Corresponding Author Keitaro Tanoi [email protected] orcid.org/0000-0001-7545-2771 Graduate School of Agricultural and Life Sciences, The University of Tokyo, 1-1-1, Yayoi, Bunkyo-ku, Tokyo, 113-8657 JapanAuthors for correspondence: emails [email protected] (KS); [email protected] (KT); [email protected] (KK)Search for more papers by this authorKoichi Kobayashi, Corresponding Author Koichi Kobayashi [email protected] orcid.org/0000-0003-3726-4790 Department of Biology, Graduate School of Science, Osaka Metropolitan University, 1-1 Gakuen-cho, Naka-ku, Sakai, Osaka, 599-8531 JapanAuthors for correspondence: emails [email protected] (KS); [email protected] (KT); [email protected] (KK)Search for more papers by this author First published: 14 February 2023 https://doi.org/10.1111/nph.18812AboutSectionsPDF ToolsRequest permissionExport citationAdd to favoritesTrack citation ShareShare Give accessShare full text accessShare full-text accessPlease review our Terms and Conditions of Use and check box below to share full-text version of article.I have read and accept the Wiley Online Library Terms and Conditions of UseShareable LinkUse the link below to share a full-text version of this article with your friends and colleagues. Learn more.Copy URL Orchidaceae is one of the most diverse families of flowering plants, with > 28 000 known species distributed among 763 genera (Christenhusz & Byng, 2016). The impressive diversity of orchids is partly linked to their epiphytic habits, which require adaptation to a dry environment (Silvera et al., 2009). Consequently, many epiphytic orchids adapt to water-limited environments by developing velamen, a multiple-layered sponge-like epidermis consisting of dead cells (Porembski & Barthlott, 1988), and utilizing the crassulacean acid metabolism (CAM) pathway (Silvera et al., 2005, 2009). Velamen plays key roles in protecting against UV radiation and in absorbing moisture and nutrients (Benzing et al., 1983; Zotz & Winkler, 2013). The CAM pathway, involving the temporal separation of CO2 fixation by phosphoenolpyruvate carboxylase (PEPC; dark cycle) and the decarboxylation of organic acids to release CO2 for refixing by RuBisCO (light cycle), reduces water loss through stomatal openings during the day (Ting, 1985). Nonetheless, the CAM properties of their leaves do not necessarily ensure CAM photosynthesis in their roots (Benzing & Ott, 1981; Goh et al., 1983; Martin et al., 2010), although aerial roots (hereafter referred to as roots) of epiphytic orchids contain chlorophyll and perform photosynthesis (Goh et al., 1983; Martin et al., 2010). For example, Benzing & Ott (1981) reported that nocturnal CO2 uptake occurs only in the roots of one of the nine orchid species with CAM leaves, namely Phalaenopsis taenialis. By contrast, CAM photosynthesis with nocturnal carbon (C) fixation occurs in the roots of several leafless epiphytic orchids, such as in the genera Campylocentrum, Chiloschista, and Polyradicion belonging to the tribe Vandeae (Benzing & Ott, 1981; Cockburn et al., 1985; Winter et al., 1985). Because of the extreme reduction in leafless epiphytic orchid shoots, these species are often referred to as shootless orchids, and their reduced shoots contribute little to the C gain (Benzing & Ott, 1981). Given that: the roots represent the only autotrophic organs in leafless epiphytic orchids; and CAM photosynthesis is conducted in the roots without stomatal regulation of gas exchange, the roots of leafless epiphytic orchids are probably specialized for photosynthesis (Roth-Nebelsick et al., 2021). Anatomical investigation of the roots strongly suggested that the unique structures, known as aeration units, including pneumathodes (nonwettable white regions of the velamen radicum), exodermal aeration cells (EACs), and specialized cortical cells (SCCs), facilitate gaseous exchange between the atmosphere and the internal gas phase of the plant in certain leafless epiphytic genera, including Campylocentrum, Dendrophylax, Microcoelia, and Polyradicion (Benzing et al., 1983; Carlsward et al., 2006a,b; Roth-Nebelsick et al., 2021). Based on the three-dimensional structure of the aeration unit, Roth-Nebelsick et al. (2021) proposed a dehydration-triggered gap closure mechanism (driven by root shrinkage) in the leafless orchid genera Dendrophylax and Microcoelia, in which: SCCs are pushed against each other; and the EAC is squeezed shut by the exodermis. However, despite these recent advances in our understanding of the anatomical aspects (e.g. Roth-Nebelsick et al., 2021), the actual function of the photosynthetic apparatus in leafless epiphytic orchids remains largely unknown. In particular, chlorophyll fluorescence analysis has not yet been used to investigate the photosynthetic properties of these orchids. Here, we focused on the genus Taeniophyllum, which almost exclusively consists of leafless species (c. 200 species) and constitutes the majority of the leafless species in the tribe Vandeae (c. 300 species in Vandeae as a whole) (Carlsward et al., 2006b). Despite the predominance of leaflessness in Taeniophyllum and some demonstration of CAM rhythm in some other leafless epiphytic orchid genera (Cockburn et al., 1985; Winter et al., 1985), knowledge of photosynthetic properties (e.g. CO2 uptake during the night) is notably lacking in Taeniophyllum species. Fortunately, a Japanese Taeniophyllum species, T. aphyllum, often co-occurs with a leafy species Thrixspermum japonicum, which belongs to the same subtribe Aeridinae (Fig. 1a). Here, we compared the photosynthetic properties of T. aphyllum roots with those of the roots and leaves of the co-occurring species, T. japonicum. Specifically, we aimed to determine whether: T. aphyllum roots exhibit chloroplast development similar to that in T. japonicum leaves based on chloroplast ultrastructure and photosynthetic pigments; T. japonicum roots display photosynthetic electron transport activity similar to that in T. japonicum leaves based on chlorophyll fluorescence parameters; T. aphyllum roots exhibit nocturnal CO2 uptake comparable to that in T. japonicum leaves based on 14CO2 labeling experiments (Supporting Information Fig. S1), and if so, whether the roots are morphologically specialized for CAM photosynthesis based on histological characteristics of aerial roots; T. aphyllum roots accumulate malate at night, similar to T. japonicum leaves with CAM rhythms; and T. aphyllum roots exhibit photosynthetic activity linked with CAM rhythms. Through these investigations, we demonstrate that T. aphyllum roots exhibit sophisticated adaptations for optimizing photosynthetic ability. The details of the analyses and results are provided in Methods S1 and Notes S1, respectively. Fig. 1Open in figure viewerPowerPoint Photosynthetic characteristics of Thrixspermum japonicum and Taeniophyllum aphyllum. (a) T. japonicum and T. aphyllum specimens growing together (left: T. aphyllum; right: T. japonicum). Bar, 10 mm. (b) Maximum quantum yield of photosystem II (Fv/Fm) and (c) photosynthetically active light absorptivity (Abs). Different letters indicate statistically significant differences (Tukey–Kramer test: P < 0.05). (d–g) Light-response curves for the effective quantum yield of photosystem II (YII; d), quantum yield of open photosystem II under actinic light (Fv′/Fm′; e), coefficient of photochemical quenching (qP; f), and electron transport rate (ETR; g) under increased actinic light intensities. Values and error bars indicate means ± SE (n = 4 for T. japonicum; n = 9 for T. aphyllum). Transmission electron microscopy showed that T. aphyllum and T. japonicum roots possess chloroplasts with highly stacked thylakoid membranes, similar to the chloroplasts observed in T. japonicum leaves (Fig. S2), suggesting that the roots of both T. japonicum and T. aphyllum function as a photosynthetic organ. Nonetheless, the content of photosynthetic pigments (chlorophylls and carotenoids) in T. japonicum roots was approximately half of that in the leaves, whereas the pigment content in T. aphyllum roots was similar to that in T. japonicum leaves (Fig. S3). The photosynthetic electron transport activity in T. japonicum roots was also lower than that in T. aphyllum roots and T. japonicum leaves (Fig. 1). Light-response curve analysis of chlorophyll fluorescence revealed that electron transport rate (ETR) was saturated under weak actinic light (55–110 μmol photons m−2 s−1) in both species (Fig. 1). This, in addition to the highly stacked thylakoid membranes (Fig. S2) and a low Chla : b ratio (Fig. S3), is a typical feature of shade-tolerant plants (Lichtenthaler et al., 1981; Ptushenko et al., 2013), whereas epiphytic orchids do not necessarily occur in habitats with low light intensities (Tsutsumi et al., 2011). Even though the ETR of T. japonicum roots decreased substantially with increasing actinic light intensity, the ETR of T. aphyllum roots remained similar to that of T. japonicum leaves, even under intense light. The coefficient of photochemical quenching (qP), which represents the redox state of the photosystem II (PSII) acceptor side, was higher in T. aphyllum roots than in T. japonicum roots. This suggests that T. aphyllum roots are likely more effective than T. japonicum roots in maintaining PSII in an oxidized state and are thus more effective in performing photosynthesis under high-light conditions. Interestingly, T. japonicum roots have denser velamen layers than T. aphyllum, resulting in stronger light reflection, as seen in its silver coloration (Fig. 1a), and lower light absorptivity (Fig. 1c). The strong light reflection by the velamen arguably protects the T. japonicum roots from excessive light absorption while probably limiting the light-harvesting ability of this species. The 14C-labeling analysis revealed that T. japonicum leaves fix CO2 at night, as do typical CAM plants, whereas its roots fix CO2 mainly during the daytime, like C3 plants (Fig. 2). These features were also consistent with their malate metabolism. In T. japonicum leaves, malate content was decreased by 95% at dusk relative to that at dawn, whereas in the roots, there was no significant difference between malate content at dawn and dusk (Fig. 3). Furthermore, in T. japonicum, the elimination of CO2 by saturating atmospheric air with N2 gas at dawn significantly decreased the effective quantum yield of PSII (YII), which represents the efficiency of photosynthetic electron transport, in roots but not in leaves (Fig. S4). The data suggest that, in T. japonicum, only the roots rely on atmospheric CO2 to drive photosynthetic electron transport at dawn, although the influence of elimination of O2 together with CO2 in this process cannot be completely ruled out. From these results, we conclude that T. japonicum performs CAM photosynthesis in leaves and C3 photosynthesis in roots. Of note, the maximal CO2-fixing activity in T. japonicum roots was lower than that in the leaves. Considering that photosynthetic pigment content and photosynthetic electron transport activity in T. japonicum roots were much lower than those in the leaves, photosynthesis in T. japonicum roots may be subsidiary to the main activity in leaves, possibly due to their inability to perform regulated gas exchange. Nevertheless, our data indicate a low but significant fixation of external CO2 in T. japonicum roots, while it has been speculated that the roots of epiphytic orchids mainly conduct effective internal refixation of respiratory CO2 (Aschan & Pfanz, 2003). Fig. 2Open in figure viewerPowerPoint Assimilation of 14CO2 by Thrixspermum japonicum and Taeniophyllum aphyllum organs under light and dark conditions. (a) Radioluminographs of plant organs (leaves and/or roots). Color bar scale shows the relative intensity of photostimulated luminescence. (b) 14C activity of plant organ samples, measured by liquid scintillation counting. Values and error bars indicate means ± SE (n = 4 or 5). Different letters indicate statistically significant differences (Tukey–Kramer test: P < 0.05). FW, fresh weight. Fig. 3Open in figure viewerPowerPoint l-Malate content of Thrixspermum japonicum and Taeniophyllum aphyllum organs at the onset (dawn) and end (dusk) of the light period. Values and error bars indicate means ± SE (n = 3). Different letters indicate significant differences (Tukey–Kramer test: P < 0.05). FW, fresh weight. By contrast, in leafless epiphytic orchids, roots are fully responsible for autotrophic C gain. Therefore, in addition to refixing respiratory CO2, their roots must also perform autotrophic functions more efficiently than those of leafy epiphytic orchids. As expected, photosynthetic pigment content and ETR in T. aphyllum roots were higher than those in T. japonicum roots. As indicated by nocturnal malate accumulation (Fig. 3), the 14CO2 feeding experiments showed that the roots of T. aphyllum primarily take up CO2 under dark conditions, which is typical of CAM plants (Fig. 2). Subsequently, similar to T. japonicum leaves, elimination of atmospheric CO2 by N2 gas did not affect the YII of T. aphyllum roots at dawn (Fig. S4), implying that T. aphyllum roots largely rely on internal CO2 stored in the form of malate during the night. To date, evidence for CAM photosynthesis has been restricted to the 13C enrichment in T. malianum (Winter et al., 1983). Our demonstration of CAM properties in T. aphyllum belonging to Taeniophyllum, the most species-rich genus of leafless epiphytic orchids, strongly suggests that the transfer of CAM photosynthesis from leaves to roots plays a crucial role in the evolution of leaflessness. Moreover, the high nocturnal C fixation by T. aphyllum roots comparable to that by T. japonicum leaves indicates sophisticated C fixation in T. aphyllum roots. It is widely acknowledged that, in the leaves of CAM plants, the rate of nocturnal CO2 fixation by PEPC is regulated by a variety of mesophyll factors, including the cellular concentration of l-malate and the expression of PEPC kinase, in addition to stomatal conductance (Borland & Taybi, 2004; Borland et al., 2011). The nocturnal enhancement of malate accumulation and CO2 uptake by T. aphyllum roots, to levels similar to those in T. japonicum leaves, suggests that the diel PEPC activity in T. aphyllum is regulated by the roots. It is likely that the unique anatomical features of T. aphyllum roots, along with the higher number of pneumathodes than in T. japonicum roots, also contribute to its high nocturnal C fixation. Specifically, the EACs in T. aphyllum roots exhibited a squeezed shape between the two SCCs, whereas the EACs and SCCs in T. japonicum roots are hardly distinguishable from unspecialized exodermal and cortex cells, respectively (Fig. S5). The data suggest the morphological sophistication of T. aphyllum aeration units, as previously observed in those of other leafless orchid genera, such as Dendrophylax and Microcoelia (Roth-Nebelsick et al., 2021; Fig. S5). Moreover, unlike T. japonicum roots, the pneumathodes of T. aphyllum roots exhibited active bubble evolution in a light-dependent manner under submerged conditions (Fig. S5; Video S1), which indicates a high photosynthetic gas exchange capacity through the pneumathodes in T. aphyllum roots, even under very wet conditions. Analysis of diel chlorophyll fluorescence dynamics revealed that in T. japonicum leaves, YII and qP remained high until the end of the light period (Fig. S6), even though the malate content decreased substantially at the end of the light period (Fig. 3). Some studies have reported that the leaves of certain CAM plants behave similarly to those of C3 plants in terms of CO2 fixation because their stomata reopen when malate reserves are exhausted toward the end of the day (Spalding et al., 1979; Cote et al., 1989; Wyka & Lüttge, 2003). Thus, T. japonicum leaves might prevent over-reduction of the plastoquinone pool by using atmospheric CO2 directly for RuBisCO carboxylation toward the end of the light period. In fact, the elimination of atmospheric CO2 by N2 gas strongly decreased YII in T. japonicum leaves at dusk (Fig. S4), suggesting that T. japonicum leaves primarily rely on atmospheric CO2 to maintain photosynthetic electron transport without malate reserves. Similar responses to the N2 saturation were observed in T. aphyllum roots; therefore, T. aphyllum roots also use atmospheric CO2, presumably through the pneumathodes, when malate reserves are exhausted at dusk. The T. japonicum roots had a different diel pattern of photosynthetic parameters and significantly lower YII than those in T. japonicum leaves and T. aphyllum roots (Fig. S6). This supports our assumption that T. japonicum roots are less sophisticated as photosynthetic organs. Overall, we can conclude that T. aphyllum roots possess characteristics commonly observed in leaves of epiphytic orchids, such as high concentration of photosynthetic pigments, high rate of photosynthetic electron transport, high capacity for photosynthetic gas exchange, and nocturnal CO2 fixation. However, as did T. japonicum roots, T. aphyllum roots showed a lower maximum quantum yield of PSII than T. japonicum leaves, whose physiological relevance (e.g. partial mycoheterotrophy) awaits further investigation (Kobayashi et al., 2021). We also note that the evolutionary scenario that led to leaflessness in epiphytic orchids is a topic of ongoing research. Further studies, such as comparisons of more phylogenetically close leafy and leafless CAM species, are needed to fully understand whether the transfer of CAM photosynthesis from leaves to roots is the most plausible explanation. Moreover, the mechanisms that control gas conductance in roots without stomatal regulation of gas exchange also deserve further investigation, given that CAM photosynthesis by roots has probably played a key role in the evolution of leaflessness in epiphytic orchids. Acknowledgements We thank Marc-André Selosse and three anonymous reviewers for their constructive comments on the manuscript. We also thank Michiko Ishida, Ayako Takahashi, and Haruka Aoki for their technical assistance. This study was financially supported by the Japan Science and Technology Agency PRESTO program (grant no. JPMJPR21D6; KS) and the Japan Society for the Promotion of Science (JSPS) Grants-in-Aid for Scientific Research (KAKENHI; grant no. 22H05076; KK and NN). Competing interests None declared. Author contributions KS and KK designed the project. KS collected the materials. KK and AY conducted the experiments on photosynthetic properties. KT and RS performed the radioisotope experiments. KA, NN, KK, KS, and HO conducted the histological experiments. KS and KK wrote the initial draft with input from KT and NN. All the authors revised the manuscript and approved the final version of the manuscript. Open Research Data availability Additional supporting information is available online in the Supporting Information section at the end of the article. Supporting Information Filename Description nph18812-sup-0001-Supinfo.pdfPDF document, 12.2 MB Fig. S1 Overview of 14CO2 experiment to assay carbon assimilation. Fig. S2 Transmission electron microscopy images of plastids from Thrixspermum japonicum and Taeniophyllum aphyllum. Fig. S3 Photosynthetic pigment content of Thrixspermum japonicum and Taeniophyllum aphyllum. Fig. S4 Effects of CO2 elimination by N2 gas on effective quantum yields of photosystem II (YII) at dawn and dusk. Fig. S5 Pneumathodes and aeration units of Thrixspermum japonicum and Taeniophyllum aphyllum roots observed in wet conditions. Fig. S6 Dynamics of photosynthetic parameters in Thrixspermum japonicum and Taeniophyllum aphyllum organs over a 10 h light period. Methods S1 Methods used to investigate the photosynthetic properties of Taeniophyllum aphyllum roots as well as the leaves and roots of the co-occurring species, Thrixspermum japonicum. Notes S1 Photosynthetic properties of Taeniophyllum aphyllum roots and Thrixspermum japonicum leaves and roots. nph18812-sup-0002-VideoS1.mp4MPEG-4 video, 1.5 MB Video S1 Evolution of a bubble from a white spot (pneumathode) on the Taeniophyllum aphyllum root. Please note: Wiley is not responsible for the content or functionality of any Supporting Information supplied by the authors. Any queries (other than missing material) should be directed to the New Phytologist Central Office. Please note: The publisher is not responsible for the content or functionality of any supporting information supplied by the authors. Any queries (other than missing content) should be directed to the corresponding author for the article. References Aschan G, Pfanz H. 2003. Non-foliar photosynthesis – a strategy of additional carbon acquisition. Flora 198: 81– 97. Benzing DH, Friedman WE, Peterson G, Renfrow A. 1983. Shootlessness, velamentous roots, and the pre-eminence of Orchidaceae in the epiphytic biotope. American Journal of Botany 70: 121– 133. Benzing DH, Ott DW. 1981. Vegetative reduction in epiphytic Bromeliaceae and Orchidaceae: its origin and significance. Biotropica 13: 131– 140. Borland AM, Barrera Zambrano VA, Ceusters J, Shorrock K. 2011. The photosynthetic plasticity of crassulacean acid metabolism: an evolutionary innovation for sustainable productivity in a changing world. New Phytologist 191: 619– 633. Borland AM, Taybi T. 2004. Synchronization of metabolic processes in plants with crassulacean acid metabolism. Journal of Experimental Botany 55: 1255– 1265. Carlsward BS, Stern WL, Bytebier B. 2006a. Comparative vegetative anatomy and systematics of the angraecoids (Vandeae, Orchidaceae) with an emphasis on the leafless habit. Botanical Journal of the Linnean Society 151: 165– 218. Carlsward BS, Whitten WM, Williams NH, Bytebier B. 2006b. Molecular phylogenetics of Vandeae (Orchidaceae) and the evolution of leaflessness. American Journal of Botany 93: 770– 786. Christenhusz MJM, Byng JW. 2016. The number of known plants species in the world and its annual increase. Phytotaxa 261: 201– 217. Cockburn W, Goh CJ, Avadhani PN. 1985. Photosynthetic carbon assimilation in a shootless orchid, Chiloschista usneoides (Don) Ldl: a variant on crassulacean acid metabolism. Plant Physiology 77: 83– 86. Cote FX, Andre M, Folliot M, Massimino D, Daguenet A. 1989. CO2 and O2 exchanges in the CAM plant Ananas comosus (L.) Merr: determination of total and malate-decorboxylation-dependent CO2-assimilation rates; study of light O2-uptake. Plant Physiology 89: 61– 68. Goh CJ, Arditti J, Avadhani PN. 1983. Carbon fixation in orchid aerial roots. New Phytologist 95: 367– 374. Kobayashi K, Suetsugu K, Wada H. 2021. The leafless orchid Cymbidium macrorhizon performs photosynthesis in the pericarp during the fruiting season. Plant and Cell Physiology 62: 472– 481. Lichtenthaler HK, Buschmann C, Döll M, Fietz H-J, Bach T, Kozel U, Meier D, Rahmsdorf U. 1981. Photosynthetic activity, chloroplast ultrastructure, and leaf characteristics of high-light and low-light plants and of sun and shade leaves. Photosynthesis Research 2: 115– 141. Martin CE, Mas EJ, Lu C, Ong BL. 2010. The photosynthetic pathway of the roots of twelve epiphytic orchids with CAM leaves. Photosynthetica 48: 42– 50. Porembski S, Barthlott W. 1988. Velamen radicum micromorphology and classification of Orchidaceae. Nordic Journal of Botany 8: 117– 137. Ptushenko VV, Ptushenko EA, Samoilova OP, Tikhonov AN. 2013. Chlorophyll fluorescence in the leaves of Tradescantia species of different ecological groups: induction events at different intensities of actinic light. Biosystems 114: 85– 97. Roth-Nebelsick A, Thiv M, Malkowsky Y, Schott R, Heyer A. 2021. Structure and functional anatomy of the gas exchange apparatus of leafless orchids: evidence for a control mechanism? Botanical Journal of the Linnean Society 197: 249– 262. Silvera K, Santiago LS, Cushman JC, Winter K. 2009. Crassulacean acid metabolism and epiphytism linked to adaptive radiations in the Orchidaceae. Plant Physiology 149: 1838– 1847. Silvera K, Santiago LS, Winter K. 2005. Distribution of crassulacean acid metabolism in orchids of Panama: evidence of selection for weak and strong modes. Functional Plant Biology 32: 397– 407. Spalding MH, Stumpf DK, Ku MSB, Burris RH, Edwards GE. 1979. Crassulacean acid metabolism and diurnal variations of internal CO2 and O2 concentrations in Sedum praealtum DC. Functional Plant Biology 6: 557– 567. Ting IP. 1985. Crassulacean acid metabolism. Annual Review of Plant Physiology 36: 595– 622. Tsutsumi C, Miyoshi K, Yukawa T, Kato M. 2011. Responses of seed germination and protocorm formation to light intensity and temperature in epiphytic and terrestrial Liparis (Orchidaceae). Botany 89: 841– 848. Winter K, Medina E, Garcia V, Luisa Mayoral M, Muniz R. 1985. Crassulacean acid metabolism in roots of a leafless orchid, Campylocentrum tyrridion Garay & Dunsterv. Journal of Plant Physiology 118: 73– 78. Winter K, Wallace BJ, Stocker GC, Roksandic Z. 1983. Crassulacean acid metabolism in australian vascular epiphytes and some related species. Oecologia 57: 129– 141. Wyka TP, Lüttge UE. 2003. Contribution of C3 carboxylation to the circadian rhythm of carbon dioxide uptake in a crassulacean acid metabolism plant Kalanchoë daigremontiana. Journal of Experimental Botany 54: 1471– 1479. Zotz G, Winkler U. 2013. Aerial roots of epiphytic orchids: the velamen radicum and its role in water and nutrient uptake. Oecologia 171: 733– 741. Volume238, Issue3May 2023Pages 932-937 FiguresReferencesRelatedInformation
MoreTranslated text
Key words
(CO2)-C-14 labeling,carbon acquisition,chlorophyll fluorescence,crassulacean acid metabolism (CAM),Orchidaceae,radioisotope
AI Read Science
Must-Reading Tree
Example
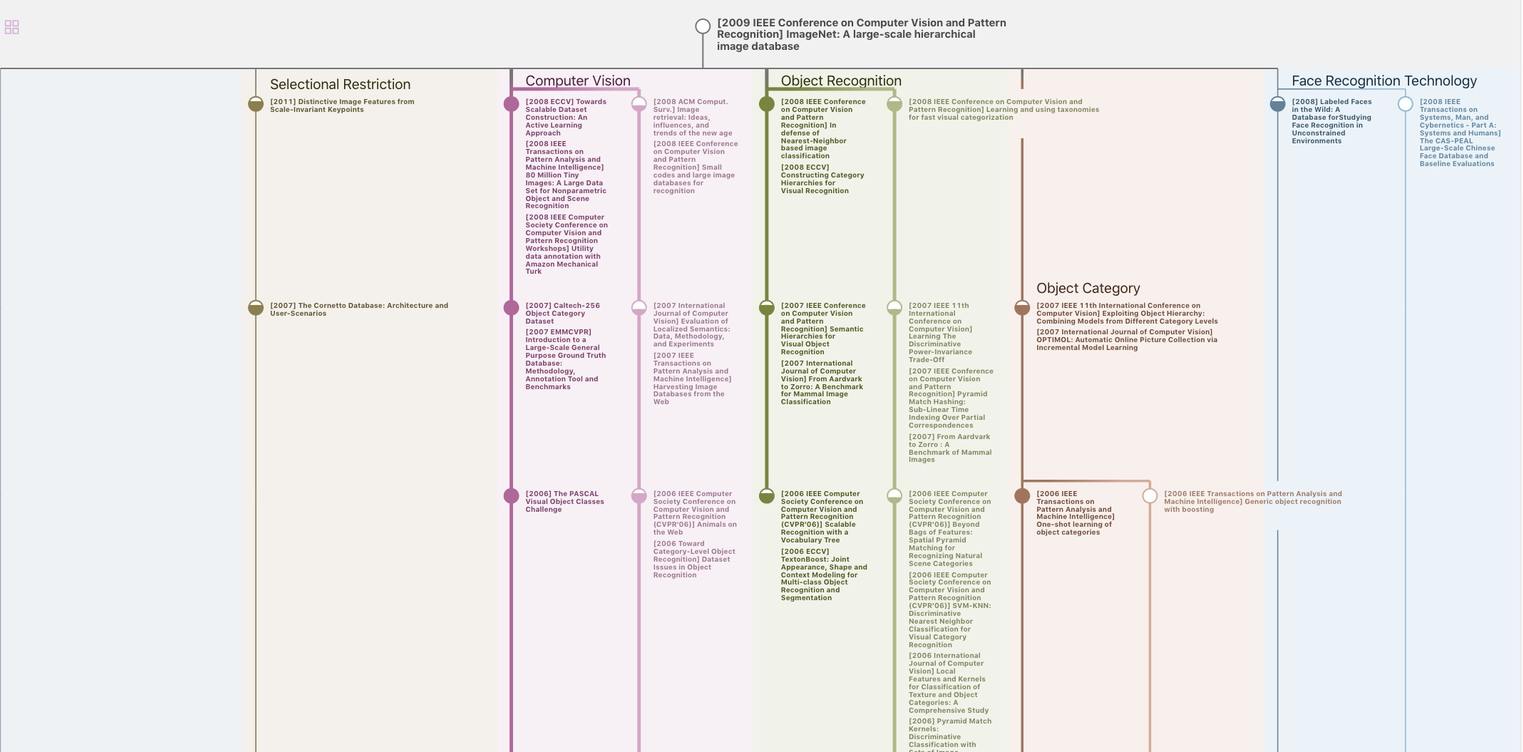
Generate MRT to find the research sequence of this paper
Chat Paper
Summary is being generated by the instructions you defined