Greenhouse Gas Fluxes from the Falmouth Wastewater Treatment Facility and Implications for Groundwater Entering West Falmouth Harbor
semanticscholar(2017)
摘要
Nitrous oxide production is an inherent consequence of biological nitrogen removal with the use of a Sequencing Batch Reactor (SBR). We sought to quantify the flux of nitrous oxide and methane associated with a single SBR cycle at the Falmouth Wastewater Treatment Facility using a temporally accurate approach. Aerobic sequences were primarily responsible for nitrous oxide and methane release, though the largest flux of nitrous oxide would occur outside of the SBR, as a result of effluent equilibration with the atmosphere. The observed cycle had a nitrous oxide emission factor of 0.36%, which is consistent with other similar operations. There is already evidence of a reduction in nitrogen loading to West Falmouth Harbor as a result of SBR installation. Introduction Wastewater treatment is imperative for removing excess anthropogenic nitrogen before it can enter nutrient-sensitive water bodies, leading to ecosystem damage (Anderson et al., 2002). In an attempt to reduce the nutrient flux to the nearby West Falmouth Harbor, the city of Falmouth installed new equipment to their wastewater treatment facility (WWTF) in late 2005 (Figure 1). The addition of two sequencing batch reactors (SBRs) in 2005 helped the WWTF reduce effluent nitrogen content (Figure 2). The sequencing batch reactors promote biological removal of nitrogen from wastewater in two steps. First, the influent is subjected to a six-part oxygenation series, followed by a quiescent stage during which the reactor goes anoxic. The aerobic stages promote microbial breakdown of nitrogencontaining dissolved organic material as well as microbial nitrification. The anaerobic stage provides conditions for denitrifying bacteria to convert dissolved nitrate (NO3-) to harmless dinitrogen gas (N2), thus removing nitrate from the water (Figure 3). A consequence of biological nitrogen removal is the release of nitrous oxide (N2O) as a by-product (Mørkved et al., 2007). N2O is a greenhouse gas approximately 300 times more potent than carbon dioxide (CO2), and additionally depletes the stratospheric ozone (O3) layer (IPCC, 2013 and Portmann et al., 2012). N2O is the final intermediate in denitrification, and nitrification can also be a source of N2O, thus both cycles of the SBR could be responsible for N2O emissions. We collected a temporally accurate sequence of GHG emission from the SBRs, and then substantiated this data with water quality measurements in order to understand the conditions that elicit greenhouse gas production. The dataset allowed us to identify emissions trends within the sequence for methane (CH4), carbon monoxide (CO), CO2, and most importantly N2O. We used these measurements to estimate annual emissions data for the SBRs as well as their N2O emission factor (the ratio of moles N2O-N released per mole nitrogen load). At West Falmouth Harbor, we collected a series of groundwater samples and investigated trends in nitrogen species, depth, and location relative to the known WWTF effluent plume (Moseman-Valtierra et al, 2015). We then sought to identify any effect of the addition of SBRs to the WWTF on groundwater quality entering the harbor. Methods We collected data from two sites: The Falmouth Wastewater Treatment Facility and the shores of West Falmouth Harbor. The Los Gatos Research Ultraportable Greenhouse Gas Analyzer (CH4, CO2 and H2O) and N2O/CO-23r were available for measuring gas concentrations in a connected chamber. The LGRs are traditionally used with chambers that sit on solid surfaces, thus the chamber employed for this study had to float steadily in order to make analogous measurements. The analysis of GHG emission at the WWTF presented some additional difficulties the goal of making real-time measurements in light of hazardous and varying reactor conditions (i.e. the turbulence generated by blowers during the aerobic phase, Figure 4) required a that the engineered design be able to withstand unfavorable conditions and have flexible measurement options. Thus, we selected a chamber style that allowed for aerobic and anaerobic measurements to be made with independent methodologies. Of an assortment of available materials, we found that the top section of a 55gallon drum had the ideal properties to satiate the dynamic needs of the chamber (Figure 5). The chamber needed to float at a consistent depth, such that the volume above the water contained by the chamber was constant. To achieve this, nine plastic bottles were capped at even pressure and attached to the rim of the chamber in an even distribution (every 40 degrees), thus achieving equal flotation. Flotation tests revealed that the chamber floated without any tilt, thus the trapped volume is roughly cylindrical. Thus the internal volume was calculated as the sum of the barrel cap's volume (measured with known volumes of water) and a cylinder (total chamber volume = 31.94 liters). Two bulkhead unions attached to the top of the chamber allowed us to run the LGR quarter inch input and output tubing to a splitter, such that the two LGRs could be run in parallel (Figure 6). Since the LGRs are damaged from water entering the machine, we inserted a stoppered Erlenmeyer flask on the input line to act as a fallback water trap. The chamber's removable white plastic caps made it ideal for the aerobic stage measurements, as the chamber needed to ventilate in order to maintain constant pressure. The WWTF collects data on the volume of air their blowers flush through the reactor every thirty seconds, which allowed us to calculate the flux of any gas as in Equation 1. In order to make this calculation, we had to assume even gas emission at all points along the water's surface. This likely introduces some error into the experiment, but the shear amount of turbulence caused by the array of blowers did appear to have a mostly homogenous appearance (Figures 1 and 3). Anaerobic stage measurements proved much simpler. With both caps screwed in, the chamber had a known volume of trapped air and known surface area of water covered. This allowed for gas flux and volume calculations based on the measured chamber concentrations alone, as in Equation 2. We collected 15 water samples taken throughout the sequence in gas-tight bottles, to each of which 3 mL of 50% ZnCL2 solution was added to inactivate microbial activity. We analyzed the samples for ammonium, nitrate, total dissolved nitrogen (TDN), and both dissolved CH4 and N2O. Colorimetric analysis yielded ammonium and nitrate data (Solórzano, 1969 and Griess, 1879) We chemically oxidized small subsamples, thus we could measure the nitrate in these subsamples as an analog for TDN (Bronk, 2002). We made dissolved GHG measurements using a modified headspace equilibration technique with 60 mL gas-tight syringes (Roper et al., 2012 and Magen et al., 2014). We used two gas chromatographs, one calibrated for measuring N2O and the other for CH4, to measure gas concentrations using subsamples of the same equilibrate headspace. The four highest N2O readings exceeded our standard curve, thus we were forced to extrapolate these values, decreasing the efficacy of these measurements (Figure 12). Additionally, there appears to have been substantial degassing of the wastewater while we had the chamber removed for recalibration. This is unfortunate, as we missed the opportunity to measure this flux and add to our measured total we will use the dissolved values to approximate what this flux may have been. At West Falmouth Harbor, we sampled an array of groundwater wells in and around a known wastewater plume and processed the samples identically to those from the WWTF (Figure 7). We took special care to draw the wells slowly to minimize degassing. At five sites, we collected a total of 19 samples, drawing from different depths in the aquifer.
更多查看译文
AI 理解论文
溯源树
样例
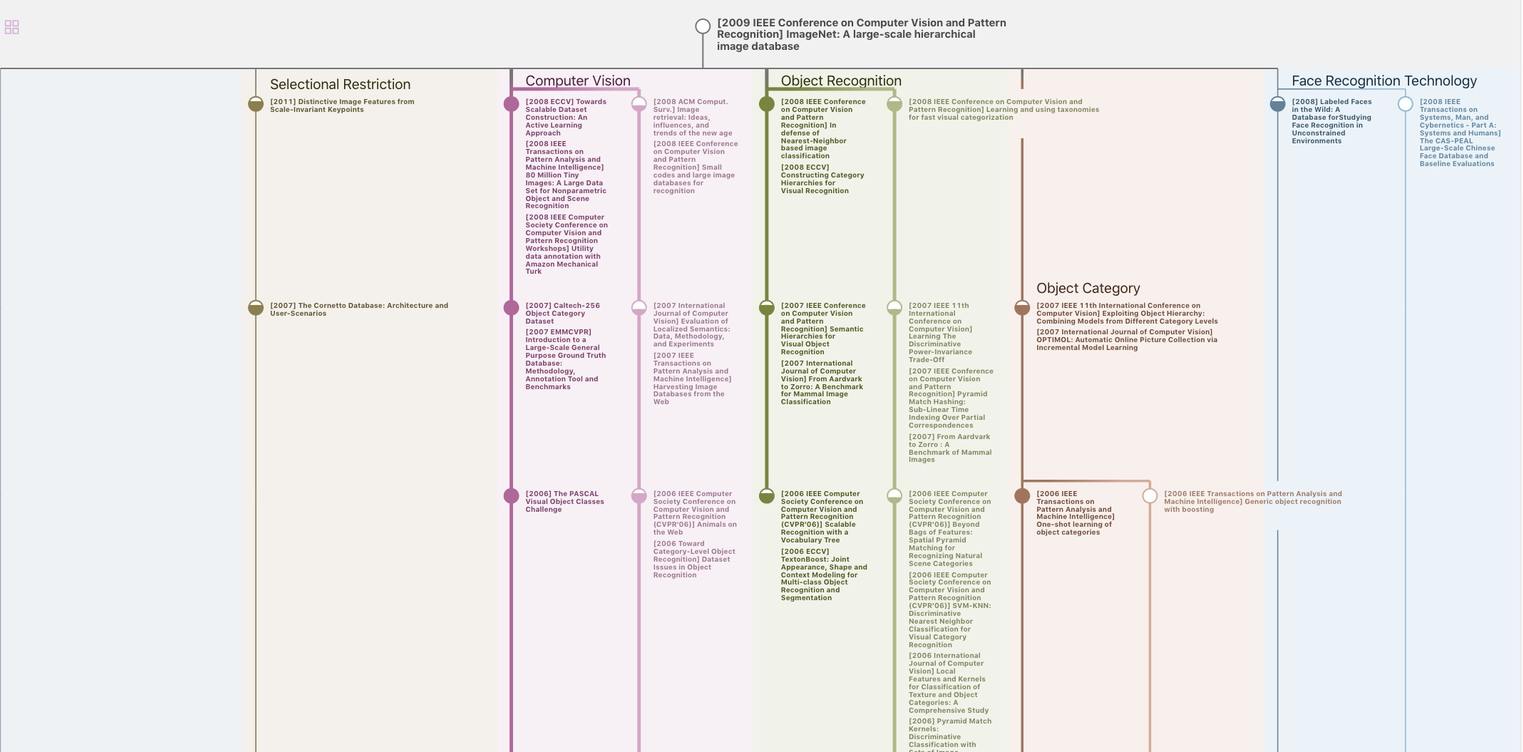
生成溯源树,研究论文发展脉络
Chat Paper
正在生成论文摘要