Fabrication of (K,Na)NbO3 glass–ceramics and crystal line patterning on glass surface
Optical Materials(2011)
摘要
Research highlights ► Crystallization behavior of (30− x )K 2 O– x Na 2 O–25Nb 2 O 5 –45SiO 2 glass were clarified. ► (K, Na)NbO 3 glass–ceramics were synthesized. ► (K, Na)NbO 3 crystal were patterned by laser-induced crystallization technique. Abstract Crystallization behavior of (30− x )K 2 O– x Na 2 O–25Nb 2 O 5 –45SiO 2 (KNNS; x = 0, 5, 10, 20 and 30) (mol%) glasses was clarified and perovskite-type nonlinear optical (K, Na)NbO 3 (KNN) crystals were synthesized by using a conventional glass–ceramics method. It was found that Na 2 O amounts over around x = 10 mol% were necessary to form perovskite-type KNN crystals showing second-harmonic generations. The substitution of K + and Na + ions was confirmed from X-ray diffraction (XRD) analysis. A continues-wave of Yb:YVO 4 fiber laser (wavelength: 1080 nm) was irradiated onto CuO doped KNNS; x = 10 (Cu–KNNS) surface. The absorption coefficient of this Cu–KNNS glass was determined to be α = 5.0 cm −1 . Perovskite-type KNN crystals were patterned in the condition of the laser power of >1.20 W and the laser scanning speed of S = 7 μm/s, and their structure was determined by Raman scattering spectra and XRD analysis. Keywords Glass Crystallization Laser patterning (K, Na)NbO 3 1 Introduction Perovskite-type alkali metal niobium oxide crystals such as lithium niobate LiNbO 3 (LN), sodium niobate NaNbO 3 (NN), or potassium niobate KNbO 3 (KN) were known as nonlinear optical materials. KN crystal is a well known perovskite-type ferroelectric or nonlinear optical materials having various attractive properties such as large second-harmonic generations (SHGs), electro-optic (EO) coefficients, and electro-mechanical coupling factors. And this crystal does not contain toxic elements like Pb in Pb(Zr, Ti)O 3 (PZT) crystal. However, it is known that the synthesis of KN ceramics and bulk single crystals are very difficult, because of, for example, a volatilization of K 2 O component during powder sintering process and an incongruent melting in the phase diagram of the K 2 O–Nb 2 O 5 system [1–3] . In addition, in the crystallization of KN crystal from glass, the metastable (not perovskite-type) KN phase is produced at lower temperature than stable (perovskite-type) KN phase [4–6] . Also it is known that KN single crystal has a problem in reliability, e.g., the easy crack formation due to the plurality phase transitions. Recent years, crystallization of glass caused by laser irradiations has been regarded as a process for spatially selected crystallization in glass [7–11] . This is called laser-induced crystallization technique. Crystal lines such as nonlinear optical β-BaB 2 O 4 [8] , LiNbO 3 [12] , Li + ion conducting LiFePO 4 [13] , photo-luminescence Er 3+ -doped CaF 2 [14] , ferroelastic β′-Gd 2 (MoO 4 ) 3 [15] , semi-conducting ZnO [16] , and strongly-correlated electron system La 0.7 Sr 0.3 MnO 3 [17] have been patterned successfully on the glass surface. In our previous study [6] , the fabrication of KN glass–ceramics was succeeded by glass composition designing, but homogeneous single phase KN crystal lines were not obtained in a laser-induced crystallization technique. On the other hand, LN or NN crystals are formed from congener alkali metal ions such as Li + or Na + . These crystals can sinter easily, and there are no metastable phases in the case of crystallization of glass. In recent years, by this background, some studies have been reported to obtain KN crystals by co-doping alkali metal ions as (K, Na)NbO 3 [18–23] . Wang et al. [18] tried to fabricate high dense (Na 1− X K X )NbO 3 ( X = 0.5–0.7) ceramics by using a spark-plasma-sintering (SPS) method and compared with a sample made by a hot-press method. Soderlind et al. [19] reported the fabrication and characterization of Na 0.5 K 0.5 NbO 3 thin films formed by three different sol–gel methods. Du et al. [20] reported the preparation of (Na 0.5 K 0.5 )NbO 3 ceramics by changing heat-treatment conditions without any pressure assist. Fisher et al. [21] reported (K 0.5 Na 0.5 )NbO 3 single crystals synthesized by solid state reaction with thickness up to 160 μm, in which (K 0.5 Na 0.5 )NbO 3 single crystals have been grown on the (0 0 1) surface of KTaO 3 single crystal substrate. Also, Fisher et al. [22] fabricated (K 0.5 Na 0.5 )NbO 3 ceramics in O 2 , N 2 , 75N 2 –25H 2 (mol%), and air atmosphere. Tellier et al. [23] researched the crystal structure and phase transition of K X Na 1− X NbO 3 ( X = 0.4–0.6) and analyzed by Rietveld method. It should be noted that the almost K and Na ratio of their co-doped researches have a concentration around K:Na = 1:1. This ratio has an effect as morphotropic phase boundary (MPB). Due to this effect, the problem in KN crystals exhibiting easy crack formation can reduce with keeping the KN properties as high Curie-temperature, high piezoelectric constant and so on. In order to fabricate crystallized glasses with perovskite-type nonlinear optical crystals such as KNN and desired morphologies, further studies on the formation of KNN crystals in various glasses are necessary. In the present study, we focused our attention on the formation of perovskite-type KNN crystals in K 2 O–Na 2 O–Nb 2 O 5 –SiO 2 glasses and laser patterning of KNN crystal lines on the glass surface. In particular, the crystallization behavior controlled by K:Na ratio in (30− x )K 2 O– x Na 2 O–25Nb 2 O 5 –45SiO 2 glasses are examined. 2 Experimental The glasses with the nominal compositions (30− x )K 2 O– x Na 2 O–25Nb 2 O 5 –45SiO 2 (KNNS; x = 0, 5, 10, 20 and 30) (mol%), and 20K 2 O–10Na 2 O–25Nb 2 O 5 –43SiO 2 –2CuO (Cu–KNNS) (mol%) were prepared by using a conventional melt-quenching method. In order to form crystal lines by laser-induced crystallization technique, CuO (laser absorbers) containing glass was made. Raw materials K 2 CO 3 , Na 2 CO 3 , Nb 2 O 5 , SiO 2 , and CuO were mixed and melted in a platinum crucible at 1500 °C for 1 h in air in an electric furnace. The melts were poured onto an iron plate heat-assisted at 200 °C and pressed to a thickness about 1 mm with another iron plate. The glass transition ( T g ) and crystallization peak ( T p ) temperatures were determined by using a differential thermal analysis (DTA) at a heating rate 10 K/min. To eliminate the residual internal stresses, the as-quenched glass samples were annealed (not to crystallization) at T g for 30 min in air. The glass samples were mechanically polished to a mirror finish with CeO 2 powders. Several KNNS glass samples were heat-treated at crystallization temperatures for 5 h in air, and the crystalline phases in heat-treated glass samples were identified from X-ray diffraction (XRD) analyzes (Cu Kα radiation, λ = 0.154056 nm) and Raman scattering spectrum measurements. SHGs of crystallized glass samples were examined by measuring second-harmonic waves λ = 532 nm) for the incident light of a Q -switched Nd:YAG laser with λ = 1064 nm. Optical absorption spectra at room temperature for the KNNS; x = 10 and Cu–KNNS glasses were measured in the wavelength range from 300 to 2000 nm by using a spectrometer (Shimadzu U-3120). Cw–Yb:YVO 4 fiber lasers ( λ = 1080 nm) were irradiated onto the surface of polished Cu–KNNS glass using an objective lens (magnification: 50 times, numerical aperture: NA = 0.8). The glass sample was put on the stage and mechanically moved during laser irradiations to pattern crystals. Laser powers ( P ) of P = 1.10–1.25 W and scanning speeds ( S ) of S = 7 μm/s were chosen. Morphologies and crystalline phases of laser irradiated parts were examined from polarized microscope observations, laser microscope observations, and Raman scattering spectrum measurements. And 130 lines were patterned into the 16 mm 2 (4 mm × 4 mm) surface with P = 1.20 W and S = 7 μm/s for XRD analysis. 3 Results and discussion 3.1 Fabrication of glass and glass–ceramics All glasses prepared in this study showed only halo patterns in XRD patterns. The DTA curves for the bulk glass samples of KNNS; x = 0–30 are shown in Fig. 1 . Endothermic peaks due to the glass transition and exothermic peaks due to the crystallization peak temperatures are observed. The KNNS; x = 0 shows the values of T g = 647 °C, T p1 = 748 °C, T p2 = 770 °C and T p3 = 827 °C; KNNS; x = 5 shows the values of T g = 624 °C, T p1 = 764 °C, T p2 = 852 °C and T p3 = 877 °C; KNNS; x = 10 shows the values of T g = 616 °C and T p = 754 °C; KNNS; x = 20 shows the values of T g = 622 °C and T p = 749 °C; KNNS; x = 30 shows the values of T g = 640 °C and T p = 739 °C. It is noted that KNNS; x = 0 and x = 5 glasses showed plural crystallization peaks, i.e., T p1 , T p2 and T p3 appearing in DTA patterns. On the other hand, KNNS; x = 10 to x = 30 glasses showed single crystallization peak. These results imply that the glasses with x = 10, 20 and 30 mol% might show single crystalline phase after heat-treatment of these glasses. It is also seen that the values of T g increased with the substitution of Na 2 O with K 2 O in the composition of KNNS; x = 10–30, but contrary the vales of T p decreased with this substitution. And the sharpness and height of these crystallization peaks increased with the increase of Na 2 O content. It is suggested from these results that the crystallization of KNNS; x = 30 is easier than that of the glass with x = 10 composition. KNNS glasses were heat-treated at several crystallization peak temperatures for 5 h in air, and their XRD patterns at room temperature are shown in Fig. 2–4 (orthorhombic KNbO 3 ; ICDD No.: 000320822, orthorhombic NaNbO 3 ; ICDD No.: 00031270). Fig. 2 shows the XRD patterns for the KNNS; x = 0 sample heat-treated at three different temperatures corresponding to T p1 , T p2 and T p3 . It is found that KNbO 3 crystals are not formed. The XRD peaks shown in Fig. 2 were not identified at this moment. Fig. 3 shows XRD analysis of KNNS; x = 5 and the results are similar to the data from the previous samples. Fig. 4 shows the XRD patterns for the KNNS; x = 10, 20 and 30. In the KNNS; x = 10 and x = 20 samples, only the formation of KNN crystals is detected. And in the KNNS; x = 30 glass, it is found that only NaNbO 3 crystals are formed. These results, therefore, indicate that the fabrication of perovskite-type KNN glass–ceramics was succeeded by the substitution of Na 2 O for K 2 O in the glasses with the compositions of x = 10∼30. The XRD pattern of powdered KNNS; x = 10 glass–ceramics is shown in Fig. 5 . It is seen that the powder sample gives several sharp peaks corresponding to the (K, Na)NbO 3 crystalline phase. Therefore, it is concluded that the inside part of glass–ceramics is also consisted of (K, Na)NbO 3 crystals. As shown in Figs. 4 and 5 , the intensity ratio of the XRD peaks is different in the bulk (surface) and powder (inside) samples, suggesting the possibility of (K, Na)NbO 3 crystal orientation at the surface of KNNS; x = 10. On the other hand, any difference in the intensity ratio of the XRD peaks was not observed in KNNS; x = 30 glass–ceramics. So far, any crystal orientation of NaNbO 3 at the surface of crystallized silicate glasses has not been reported [24] . It is suggested that (K, Na)NbO 3 crystal orientation at the surface of KNNS; x = 10 is closely related to the presence of K 2 O. Indeed, Tanaka et al. [4] reported that the c -axis orientated KNbO 3 crystals are formed in the crystallization of K 2 O–NbO 5 –SiO 2 glasses. The SHG microscope photograph for the powdered sample obtained by a heat-treatment at the crystallization temperature for KNNS; x = 10 glass is shown in Fig. 5 . Clear SHGs were confirmed, demonstrating that (K, Na)NbO 3 crystals formed are nonlinear optical crystals. On the other hand, it was found that the KNNS; x = 0 and 5 heat-treated samples give no clear SHG, suggesting that the metastable phase formed might have a crystal structure with an inversion symmetry. Raman scattering spectra at room temperature for KNNS; x = 0–30 glass and glass–ceramics samples obtained by heat-treatments are shown in Figs. 6–8 . In the KNNS; x = 0 glass ( Fig. 6 ), the Raman spectra showed only one broad band at 876 cm −1 , but glass–ceramic samples showed several sharp peaks at ∼465, ∼625, ∼880, and ∼850 cm −1 . The XRD patterns of these samples ( Fig. 2 ) showed that there are no peaks corresponding to the KNbO 3 crystal phase. In this point of view the Raman spectra do not confirm the formation of the KNbO 3 phase and the bands observed are a typical metastable phase. Venkataraman and Komatsu [5] reported the crystallization of a metastable phase in silicate glasses. The Raman patterns observed in this study are the same as their results. It should be pointed out that the metastable phase gives a Raman bands with a strong intensity at ∼880 cm −1 . The precursor KNNS glasses were also showed broad Raman bands at ∼875 cm −1 . It is known that SiO 2 or GeO 2 based glasses containing both alkali oxide and Nb 2 O 5 show Raman bands in the region of 800–900 cm −1 attributing to NbO 6 octahedron with non-bridging oxygen and with much distortion and broad Raman bands in the region of 600–800 cm −1 attributing to less-distorted NbO 6 octahedron with no non-bridging oxygens [25–28] . Therefore, it is suggested that the distorted NbO 6 octahedron might be included in the metastable phase and also in the precursor KNNS glasses. In the KNNS; x = 5 composition samples ( Fig. 7 ), the glass sample showed only one band at 877 cm −1 such as KNNS; x = 0 glass sample. Not only the glass sample, but also glass–ceramics sample heat-treated at 764 °C ( T p1 ) showed similar Raman spectra with KNNS; x = 0 glass–ceramics. Also glass–ceramic samples heat-treated at 852 °C ( T p2 ) and 877 °C ( T p3 ) showed similar patterns. The Raman band at 878 cm −1 being typical for the metastable phase is found in the Raman spectra of heat-treated samples at 852 and 877 °C. Unfortunately, this metastable phase is not a target crystal in the present work. But, KNNS; x = 10, 20, and 30 samples heat-treated at several crystallization temperatures showed similar Raman patterns, and these are typical patterns of (K, Na)NbO 3 or NaNbO 3 crystals [29–31] . The highest peak at ∼600 cm −1 can be assigned to the symmetric stretching vibration of O–Nb–O bonds in NbO 6 octahedron. Also, these precursor glass samples showed similar patterns to KNNS; x = 0 and x = 5 compositions. 3.2 Laser patterning of (K, Na)NbO 3 crystals The optical absorption spectra at room temperature for the KNNS; x = 10 and Cu–KNNS glasses are shown in Fig. 9 . KNNS; x = 10 glass is colorless in the visible region, but Cu–KNNS glass has a green color. The green color of Cu–KNNS glass is coming from Cu 2+ ions with the electronic configuration 3d 9 giving a strong and broad absorption peak at ∼800 nm. The peak at ∼800 nm is assigned to the 2 B 1g → 2 B 2g transition in Cu 2+ ions in octahedral sites with strong tetragonal distortion [32,33] . As can be seen from Fig. 9 , the absorption edge is shifting to longer wavelength by addition of CuO. This shift would be assigned to the transition of 3d 10 → 3d 9 4s in Cu + ions [34] . From these results we can summarize that both Cu + and Cu 2+ ions are present in Cu–KNNS glass. The value of the absorption coefficient ( α ) at the wavelength of λ = 1080 nm corresponding to the wavelength of Yb:YVO 4 fiber laser is evaluated to be α = 5.0 cm −1 for Cu–KNNS glass. Yb:YVO 4 fiber laser ( λ = 1080 nm) was used to form and pattern (K, Na)NbO 3 crystals onto the KNNS x = 10 glass surface. The phenomenon taking place during laser irradiations in glasses depends on the combination of the laser type (wavelength, power, irradiation time, scanning speed) and materials type (system, composition, thermal stability against crystallization) [8,35] . We carried out laser irradiations in the conditions of laser powers of P = 1.10–1.25 W and scanning speeds of S = 7 μm/s. The polarized optical photograph for the laser irradiated Cu–KNNS sample is shown in Fig. 10 . With laser irradiation powers less than P = 1.05 W, there was no structural change at the surface. It is seen that the width of structure changed parts changes largely depending on laser power, i.e., ∼3 μm width P = 1.10 W and ∼10 μm width P = 1.25 W. The circles of the right side of crystal lines are signatures when laser scanning stopped in one position. The laser micro photograph for laser irradiated Cu–KNNS sample is shown in Fig. 11 . It is seen that the height of crystal lines become increase by increase of laser irradiation power. The height of the line becomes around 1.5 μm when P = 1.25 W and the line around 0.5 μm is produced with P = 1.10 W. In this point of view the volume of crystal lines increase by increase of laser irradiation power. The Raman scattering spectra for the laser irradiated parts at room temperature were measured and shown in Fig. 12 together with the spectrum of KNNS; x = 10 glass–ceramics sample. For the lines patterned by laser irradiations with P = 1.10 W and P = 1.15 W, the Raman spectra showed the peak at 880 cm −1 . These patterns are similar to the Raman spectra for the precursor glasses. That is, it is concluded that laser irradiations with powers less than P = 1.15 W do not cause crystallization but induce the change only in the refractive index. On the other hand, the crystal lines patterned with > P = 1.20 W show the Raman spectra assigning to typical (K, Na)NbO 3 crystals. But still weak peaks are observed at ∼880 cm −1 being a typical Raman band for glass samples. These results suggest that in the present crystallized line there are not only (K, Na)NbO 3 crystalline phase but also some glassy phase is remained. The intensity of the Raman band at ∼600 cm −1 for the crystal line patterned with P = 1.25 W is higher than that for the line patterned with P = 1.20 W. It should be pointed out, however, that the laser irradiation with P = 1.25 W induces easily the crack formation. In this point of view the crystal line patterning for XRD analysis was done at the condition of P = 1.20 W and S = 7 μm/s. The polarized optical photograph for the cross-section part of the crystal line patterned with P = 1.20 W and S = 7 μm/s is shown in Fig. 13 . It is found that there are three different regions, i.e., the crystallized part, the refractive index changed part, and the glass part. Usually, the center of the surface part becomes the highest temperature in the laser-induced crystallization technique, and the temperature of the surrounding part is becoming low, depending on the distance from the center part. It is expected that the crystallization is taking place mainly in the center part and only structural relaxation giving the change in the refractive index would be induced in the surrounding part. If the temperature of the center region is lower than the crystallization temperature of a given glass, only structural relaxation would be expected. The Raman spectra shown in Fig. 12 would indicate, therefore, that the temperature of the laser irradiated region with a condition of P = 1.15 W is lower than the crystallization temperature. The XRD pattern for the crystal lines patterned with P = 1.20 W and S = 7 μm/s is shown in Fig. 14 . It is found that the diffraction peaks shifted to the high angle side compared with KNbO 3 ICDD (orthorhombic KNbO 3 ; ICDD No.: 000320822) data. These results indicate that (K, Na)NbO 3 crystals containing both K + and Na + ions are patterned successfully on the glass surface. 4 Conclusions The crystallization behavior of (30− x )K 2 O– x Na 2 O–25Nb 2 O 5 –45SiO 2 (KNNS; x = 0, 5, 10, 20 and 30) (mol%) glasses was examined to synthesize perovskite-type nonlinear optical (K, Na)NbO 3 (KNN) crystals. It was found that Na 2 O amounts over around x = 10 mol% were necessary to form perovskite-type KNN crystals showing second-harmonic generations. A continues-wave of Yb:YVO 4 fiber laser (wavelength: 1080 nm) was irradiated onto CuO doped KNNS; x = 10 (Cu–KNNS) surface. Perovskite-type KNN crystals were patterned in the condition of the laser power of >1.20 W and the laser scanning speed of S = 7 μm/s, and their structure was determined by Raman scattering spectra and XRD analysis. Acknowledgements This work was supported by the Grant-in-Aid for Scientific Research from the Ministry of Education, Science, Sports, Culture and Technology, Japan, and partly by Program for Developing the Supporting System for Global Multidisciplinary Engineering Establishment in Nagaoka University of Technology. References [1] H. Birol D. Damjanovic N. Setter J. Am. Ceram. Soc. 88 2005 1754 [2] V.L. Kosyakov N.L. TsrKina N.A. Pylneva A.B. Meshalkin A.B. Kaplun J. Cryst. Growth 275 2005 e135 [3] H. Kimura A. Miyazaki K. Maiwa Z.X. Cheng C.V. Kannan Opt. Mater. 30 2007 198 [4] H. Tanaka M. Tamamoto Y. Takahashi Y. Benino T. Fujiwara T. Komatsu Opt. Mater. 22 2003 71 [5] B.H. Venkataraman T. Komatsu Mater. Res. Bull. 43 2008 2592 [6] K. Kioka T. Honma T. Komatsu Opt. Mater. 33 2011 267 [7] T. Honma Y. Benino T. Fujiwara T. Komatsu Appl. Phys. Lett. 88 2006 23105 [8] T. Komatsu R. Ihara T. Honma Y. Benino R. Sato H.G. Kim T. Fujiwara J. Am. Ceram. Soc. 90 2007 699 [9] B. Franta T. Williams C. Faris S. Feller M. Affatigao Phys Chem. Glasses: Eur. J. Glass Sci. Technol. B 48 2007 357 [10] Y. Dau B. Zhu J. Qiu H. Ma B. Lu B. Yu Chem. Phys. Lett. 443 2007 253 [11] P. Gupta H. Jain D.B. Williams T. Honma Y. Benino T. Komatsu J. Am. Ceram. Soc. 91 2008 110 [12] T. Honma K. Koshiba Y. Benino T. Kmatsu Opt. Mater. 31 2008 315 [13] K. Hirose T. Honma Y. Doi Y. Hinatsu T. Komatsu Solid State Commun. 146 2008 272 [14] M. Kanno T. Honma T. Komatsu J. Am. Ceram. Soc. 92 2009 825 [15] Y. Tsukada T. Honma T. Komatsu Appl. Phys. Lett. 94 2009 059901 [16] R. Nagai T. Honma T. Komatsu J. Am. Ceram. Soc. 93 2010 658 [17] K. Kioka T. Honma T. Ishibashi T. Komatsu Solid State Commun. 149 2009 1795 [18] R. Wang R. Xie T. Sekiya Y. Shimojo Mater. Res. Bull. 39 2004 1709 [19] F. Soderlind P.O. Kall U. Helmersson J. Cryst. Growth 281 2005 468 [20] H. Du Z. Li F. Tang S. Qu Z. Pei W. Zhou Mater. Sci. Eng. B131 2006 83 [21] J.G. Fisher A. Bencan J. Holc M. Kosec S. Vernay D. Rytz J. Cryst. Growth 303 2007 487 [22] J.G. Fisher D. Rout K.S. Moon S. Joong L. Kang J. Alloys Compd. 479 2009 467 [23] J. Tellier B. Malic B. Dkhil D. Jenko J. Cilinsek M. Kosec Solid State Sci. 11 2009 320 [24] M.P.F. Graca M.G.F. da Silva A.S.B. Sombra M.A. Valente Physica B 396 2007 62 [25] E.V. Kolobkava Fiz. Khim. Stekla 13 1987 352 [26] K. Fukumi S. Sakka J. Mater. Sci. 23 1988 2819 [27] A. Aronne V.N. Sigaev B. Champagnon E. Fanelli V. Califano L.Z. Usmanova P. Pernice J. Non-Cryst. Solids 351 2005 3610 [28] I. Enomoto Y. Benino T. Fujiwara T. Komatsu J. Solid State Chem. 179 2006 1821 [29] M.P.F. Graca M.G.F. da Silva M.A. Valente Solid State Sci. 11 2009 570 [30] C. Wang Y.D. Hou H.Y. Ge M.K. Zhu H. Yan J. Euro. Ceram. Soc. 29 2009 2589 [31] Y. Chang Z. Yang M. Dong Z. Liu Z. Wang Mater. Res. Bull. 44 2009 538 [32] A. Duran J.M.F. Navarro Phys. Chem. Glasses 26 1985 126 [33] R.P.S. Chakradhar B. Yosoda J.I. Rao N.O. Gopal J. Non-Cryst. Solids 352 2006 3864 [34] R. Debnath S.K. Das Chem. Phys. Lett. 155 1989 52 [35] T. Honma J. Ceram. Soc. Jpn. 118 2010 71
更多查看译文
关键词
Glass,Crystallization,Laser patterning,(K,Na)NbO3
AI 理解论文
溯源树
样例
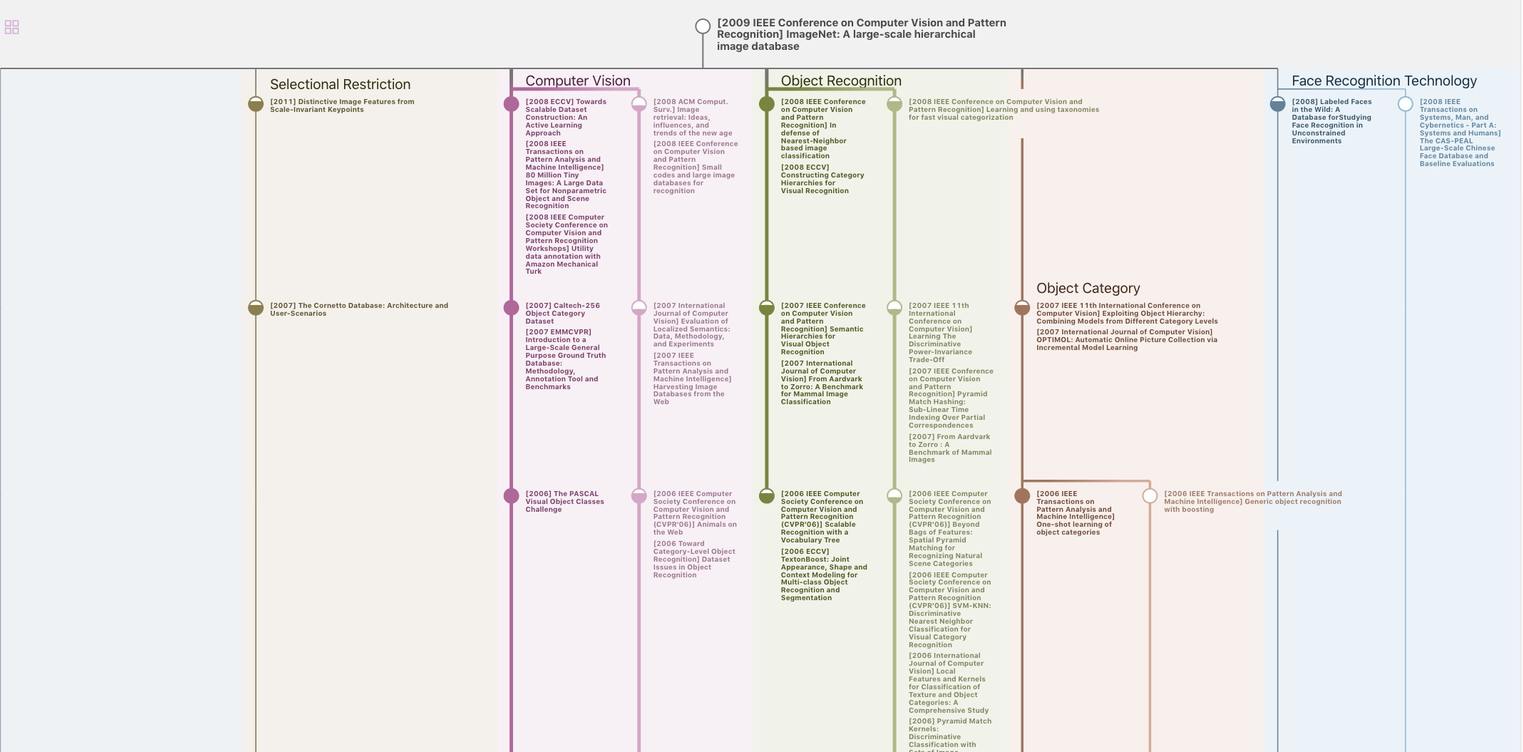
生成溯源树,研究论文发展脉络
Chat Paper
正在生成论文摘要