Effects of tempering on internal friction of carbon steels
Materials Science and Engineering: A(2011)
摘要
Two steels containing 0.626 and 0.71 wt.% carbon have been studied to determine the effects of tempering on the microstructure and the internal friction. The steels were annealed at 1093 K, quenched into water and tempered for 60 min at 423 K, 573 K and 723 K. The increase of the tempering time diminishes the martensite tetragonality due to the redistribution of carbon atoms from octahedrical interstitial sites to dislocations. Internal friction spectrum is decomposed into five peaks and an exponential background, which are attributed to the carbide precipitation and the dislocation relaxation process. Simultaneous presence of peaks P1 and P2 indicates the interaction of dislocations with the segregated carbon and carbide precipitate. Keywords Carbon steel Dislocation Internal friction Tempering 1 Introduction Steels for engineering applications are based on tempered martensitic structures. The as quenched condition represents a state of high stress, low toughness and poor ductility. After quenching, martensitic steels are tempered in order to obtain a better combination of mechanical properties. The tempering proceeds by a sequence of structural changes, including recovery of the dislocation structure and precipitation of carbides [1] . The dislocations have a strength influence on mechanical behavior, modifying the yielding and strain hardening responses. The correlation between internal friction measurements and microstructure will permit to obtain a better understanding of the strain hardening mechanism. In previous works [2,3] , correlations between the internal friction and the microstructure of two steels containing 0.626 and 0.71 wt.% carbon are proposed (A and B steels, respectively). These steels were quenched into water and tempered for 10 min at 423, 573 and 723 K. The microstructure characterization [2,3] made possible to distinguish three stages of structural changes during tempering: (I) the formation of epsilon carbide, (II) the transformation of retained austenite and (III) the formation of cementite. In both steels, the microstructure produced by the quenched was composed of a large amount of lath martensite, and small quantities of plate martensite and retained austenite [2] . The amounts of interstitial carbon in the martensitic matrix for steels A and B were estimated to be 0.40 and 0.47 wt.%, respectively, from the XRD results [3] . This suggests that carbon atoms are segregated to the strain fields of dislocations during quenching. It has been suggested [4] that dislocations and boundaries are saturated at about 0.2 wt.% C. In those works [2,3] , internal friction spectrum was decomposed into four peaks and thermal background. P1 at 215 K, P2 at 235 K, P3 at 260 K at P4 at 380 K, for 3 Hz. P1 was attributed to the interactions between edge dislocations and carbon atoms. P2 was related to the unpinning process of dislocations from carbides. P3 was related to the formation of kink pairs in the edge dislocations. P4 was associated to carbide precipitation. In this work, tempering time dependent microstructure of the two steels is studied by internal friction. The tempering time is increased to 1 h and the internal friction spectrum is compared with the results for samples tempered for 10 min that were commented in an earlier work [3] . 2 Experimental 2.5 mm × 0.5 mm × 15 mm sheets made of plain carbon steels containing 0.626 and 0.71 wt.% carbon (A and B steels, respectively) were used as test specimens. The chemical composition for both steels is listed in Table 1 . The specimens were annealed at 1093 K for 5 min in a protected atmosphere for homogenization, quenched into water and tempered for 60 min at 423, 573 and 723 K. The hardness was measured using Vickers hardness test with a load of 20 kg. The evolution of martensite lattice towards body-centered cubic during tempering was studied using X-ray diffraction (XRD). The identification of phases was realized by scanning in the region 2 θ = 20–120°. In addition, for comparing the microstructural evolution of samples tempered for 10 and 60 min, the line profiles of {1 1 0} reflections were continuously recorded in the 2 θ angular range 42–46° with stepsize of 0.026°. These measurements were performed using Cu Kα radiation ( λ = 1.542 × 10 −10 m). Internal friction measurements were performed at 3 Hz with high vacuum, in a torsion pendulum by forced vibrations method, in a temperature range from 100 to 450 K. Amplitude of deformation 3 × 10 −6 and heating rate 0.8 K/min are used. The internal friction spectrum has been deconvoluted into several Debye peaks and a thermal background. 3 Results Fig. 1 shows the hardness measurements of both steels, for quenched and tempered samples. In both steels, the hardness decreases when the tempering temperature is increased. This indicates that the dislocation density strongly dropped during tempering. Fig. 2 shows the XRD results of the quenched and tempered samples. Only peaks corresponding to martensite (α′) and austenite (γ) are visible. The peaks of the martensite phase appear in all samples. The peaks of austenite appear only in the samples quenched and tempered at 423 K. Fig. 3 shows the XRD profiles associated with the (1 1 0) reflections for the quenched and tempered samples of both steels, comparing with the results for samples tempered for 10 min that were presented in a earlier work [3] . The peak (1 1 0) shifted towards higher angles as martensite lattice evolves towards body centered cubic by the reduction of carbon content in solid solution. The tetragonality decreases more significantly with the tempering temperature than with the tempering time. The martensitic peaks of both steels are broad as a result of lattice microstraining. The microresidual stress is generated by dislocations which are produced during the quenching and by solute carbon atoms which remained in their octahedral sites without diffusion. The ferritic microstructures which form during tempering show sharper profile, their widths decrease with increasing intensity tempering. The tempering reduces the microresidual stress due to the aniquilation of dislocations and the reduction of carbon content in solid solution. Fig. 4 presents the spectrum of internal friction of the steel A, for quenched and tempered samples. The spectrum is decomposed into four peaks and a background: P1, M1, P3 and P4 at about 215, 225, 260 and 380 K, respectively. P1 and P4 only appear in the quenched sample. M1 appears in tempered samples and it is much broader than P1. In Fig. 4 , internal friction background of quenched sample is also showed. To obtain a relaxation peak, it is necessary to subtract the background thermal and a lower part of a relaxation peak at about 540 K, which is approximately an exponential as is indicated in the Debye peak [5] . According to Tkalcec et al. [6,7] , in quenched samples, there is a well-defined peak at 540 K and the background thermal increases exponentially with temperature. The background thermal is almost constant between 100 and 550 K, and it increases above 600 K. In tempered samples, the background is influenced by tempering, so the subtraction of one background has to be regarded with precaution [5] . In tempered sample at 423 K, the function of the background is similar to that of the quenched sample. In tempered samples at 573 and 723 K, internal friction background decreases, and it is considered as increasing exponentially with temperature. It is almost constant between 100 and 400 K, and then it increases. Fig. 5 shows the spectrum of internal friction of steel B for quenched sample. The spectrum is decomposed into four peaks: P1 at 215 K, P2 at 235 K, P3 at 260 K and P4 at 380 K. P1 and P2 are less broad than M1. The internal friction was measured during both the two heating processes. It has been found that P1 appears in the first heating and it disappears in the second heating. P2 only appears in the second heating. P4 only appears in the first heating due to the precipitation of epsilon carbide occurs during first heating. P3 appears in both heating. Fig. 6 shows the spectrum of internal friction of steel B, for tempered samples for 1 h at 423, 573 and 723 K. The spectrum is decomposed into three peaks: M1 at 225 K, P3 at 260 K and P5 at 428 K. P5 appears in the sample tempered at 723 K. 4 Discussion Six peaks of internal friction were observed at the following temperatures: P1 at 215 K, M1 at 225 K, P2 at 235 K, P3 at 260 K, P4 at 380 K and P5 at 428 K. Peaks P1, P2, P3 and P4 appeared in previous work on steels quenched and tempered for 10 min [3] . P1 was attributed to the interactions of the dislocations with segregated carbon [3,8,9] . P2 was associated to the interactions of the dislocations with carbide precipitates [3] . P3 was associated to the formation and migration of the thermally activated kink pairs along dislocations [3,7] . P4 was attributed to the precipitation of the epsilon carbide [3] . Liu observed an internal friction peak in Fe–Ni–C containing virgin martensite at about 215 K, which was attributed to the interaction between dislocations and carbon interstitials [8,9] . Tkalcec and Mari confirmed the essential mechanism of carbon–dislocation interactions [7] . In our previous work [2,3] , we observed two peaks at 215 and 235 K in martensitic carbon steels, which were attributed, respectively, to the interactions of dislocations with carbon atoms and carbides. As it is known, the mobility of dislocations contributes to the internal friction when the dislocation lines are unpinned from impurities or precipitates by an elastic stress [5] . The XRD results [2,3] suggest that during the quenching the carbon is segregated from interstitial sites to dislocations, and thus the dislocations are pinned by segregated carbon. As in the quenched samples of both steels P1 appears while P2 is not formed, P1 can be related to the unpinning process of dislocations from carbon segregated. In quenched sample of steel B, the first heating acts as a tempering and the carbides precipitate from segregated carbon and interstitial carbon atoms, and thus the dislocations are pinned by carbide precipitates. As during the second heating of quenched sample of steel B, P1 disappears and P2 appears, P2 can be related to the unpinning process of dislocations from carbide precipitates. The difference in peak temperatures of P1 and P2 reflects the changes in both the activation energy and the relaxation time. The breakaway of dislocation lines from the particles becomes more difficult when the proportion of carbides to segregate carbon is increased. In tempered samples for 10 min [3] , the presence of P2 indicates the interaction between dislocations and carbides. The tetragonality of the martensite is reduced with the increase of the temperature tempering by the precipitation of carbides from the segregated carbon and carbon in solid solution. The carbon segregated at dislocations disappears while epsilon carbide and cementite are precipitated. Therefore, P2 appears and P1 disappears. The tempering temperature in the tempered samples for 60 min has the same effect than that produced in the tempered samples for 10 min. The carbides are precipitated from the segregated carbon and the interstitial carbon atoms. However, the increase of the tempering time from 10 to 60 min allows the additional carbon segregation from the interstitial sites towards the dislocations as it suggest by XRD results with the evolution of (1 1 0) peak. Therefore, the dislocations interact with both carbides and segregated carbon. In tempered samples for 60 min, a broad peak M1 appears at about 225 K.This relaxation peak is much broader than a single relaxation peak. We considered this peak as a superposition of two Debye relaxation peaks, which is known as a discrete spectrum of relaxations spectrum [5] . It was necessary to introduce another peak, otherwise the fit using only Debye peaks was not satisfactory and the calculation of relaxation parameters is not reliable. M1 can be interpreted on the basis of dislocation–point defect interactions. The reason for its amplitude is that the breakaway of dislocations lines from the carbon atoms becomes easier than that from precipitates. The result is the formation of a very broad peak due to the superposition of two or more continuous relaxation peaks. Thus, a possible interpretation of broad peak is the superposition of peaks P1 and P2. Simultaneous presence of peaks P1 and P2 indicates that the pinning of the dislocations is produced by both segregated carbon and precipitates. P3 is attributed to the formation and migration of the thermally activated kink pairs along dislocations [3,7] . P3 appears in all samples of both steels. Samples tempered at 723 K have the lowest peak height. These samples have the lowest dislocation density and internal stress. It is known [8] that the higher the internal stress is, the easier the formation of the kink pairs will be. P4 only appear in the quenched samples. This peak is attributed to the precipitation of the epsilon carbide. This explication is agreed with the results during both the two heating processes of quenched sample of steel B. P4 appears during the first heating and it disappears during the second heating. It can be interpreted by a relaxation mechanism due to the direct action of the precipitates on the substructure. In tempered samples at 423 K, the rising of internal friction above 320 K is attributed to the unpinning effect of thermally activated dislocations. The precipitates formed during tempering act as a friction force to the movement of dislocations, and the dislocations can overcome this obstacle under the combined action to the applied stress and thermal energy. In quenched samples, the rising internal friction too is attributed to the unpinning effect of thermally activated dislocations. However, internal friction is increased with respect to the tempered samples at 423 K due to the easier breakaway of dislocations from the carbon atoms. Additionally, the carbide precipitation takes place during the first heating between 350 and 420 K, as it is indicated by dilatometry measurements in previous work [3] . This results in a reduction of the tetragonality of martensite lattice as it is indicated by XRD results, and thus the mobility of dislocations is increased, which contributes to the formation of P4. At temperatures above 420 K, the precipitates act as a friction force to the movement of dislocations. Precipitation hardening is detected by the decrease in the background during the second heating. It is obtained by a fine dispersion of metastable precipitates, which are able to pin the matrix dislocations. Next work correlating the height of P4 with the fraction of volume of precipitates will be presented. P5 only appears in the quenched sample at 723 K for 60 min of steel B. Some authors [10] found internal friction peaks at similar temperatures, in tempered and cold roll steels. They indicate the necessity of carbides and dislocations for the formation of peaks at this temperature. However, there is no satisfactory explanation for the relaxation mechanism and additional experimentation is required. Further works will make an attempt to use internal friction as complementary method to measure dislocation densities and activation energy of carbide precipitation in tempered martensite steels. 5 Conclusions The internal friction spectrum is decomposed into six peaks: P1 at 210 K, M1 at 225 K, P2 at 235 K, P3 at 255 K, P4 at 380 K and P5 at 428 K. P1, P2 and P3 are thermally activated mechanism, which are attributed to the dislocation relaxation process. M1 is much broader than a single relaxation peak, and this one is considered as a superposition of P1 and P2. P4 is attributed to the epsilon carbide precipitated. P5 only appears in the tempered sample at 723 K for 60 min of the steel B and it requires an additional study. The dislocation density and mobility are dependent on the intensity tempering. The increase of the tempering time reduces the martensite tetragonality due to the carbon segregation from the interstitial sites towards the dislocations. Therefore, the dislocations are pinning both by segregated carbon and carbide. P1 appears when dislocations are pinning by carbon atoms and P2 appears when dislocations are pinning by precipitates. Acknowledgements The authors would like to acknowledge the Bank of the Republic of Colombia for financial support (Project 2.042 “Internal Friction in Steels”), the “Consejo Nacional de Investigaciones Científicas y Técnicas, Comisión Nacional de Energía Atómica and Secretaría de Ciencia, Técnica y Postgrado de la Universidad Nacional de Cuyo, Argentina”. References [1] G. Krauss Metallurgical and Materials Transactions A 32 2001 861 877 [2] J.J. Hoyos, Fricción interna en aceros revenidos, Eng. Master Thesis, Universidad nacional de Colombia, 2008, pp. 30–50. [3] J.J. Hoyos A. Ghilarducci H. Salva C. Chaves J. Vélez Materials Science and Engineering A 521–522 2009 347 350 [4] T. Waterschoot K. Verbeken B.C. De Cooman ISIJ International 46 1 2006 138 146 [5] R. Schaller G. Fantozzi G. Gremaud Mechanical Spectroscopy Q-12001 2001 Trans Tech Publications pp. 71–73, 437–450 [6] I. Tkalcec D. Mari W. Benoit Materials Science and Engineering A 442 2006 471 475 [7] I. Tkalcec D. Mari Materials Science and Engineering A 370 2004 213 217 [8] Y. Liu Acta Metallurgical and Materials 42 3 1994 621 630 [9] Y. Liu Acta Metallurgical and Materials 41 11 1993 3277 3287 [10] M. Blanter I. Golovin H. Neuhäser H. Sinning Internal Friction in Metallic Materials 2007 Springer pp. 356–380
更多查看译文
关键词
Carbon steel,Dislocation,Internal friction,Tempering
AI 理解论文
溯源树
样例
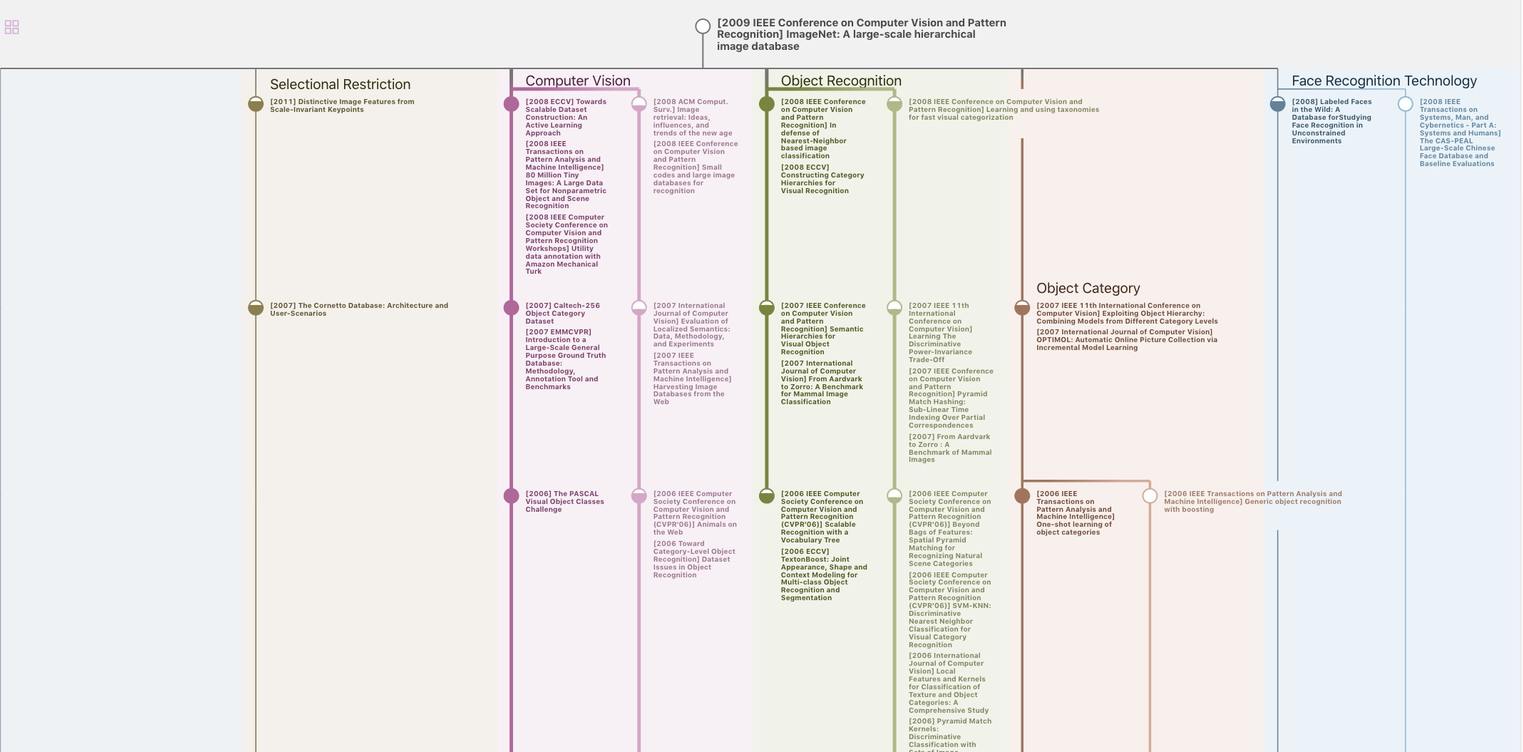
生成溯源树,研究论文发展脉络
Chat Paper
正在生成论文摘要