The biosynthesis of liposidomycin-like A-90289 antibiotics featuring a new type of sulfotransferase.
CHEMBIOCHEM(2010)
Abstract
Lip reading: The biosynthetic gene cluster for A-90289, a fatty acid nucleoside antibiotic, was cloned and sequenced. The sulfotransferase LipB is demonstrated to be essential for A-90289 biosynthesis, and in vitro characterization revealed LipB utilizes p-nitrophenylsulfate as an aryl sulfate donor and a variety of nonaryl acceptors including caprazamycin A to give the 2′-O-sulfated product, A-90289A. Enzymes involved in peptidoglycan cell wall biosynthesis are proven targets for antibacterial drugs that have revolutionized medicine.1 Remarkably, however, the majority of the enzymes involved in peptidoglycan biosynthesis have yet to be exploited in the clinic.2 Bacterial translocase I (also annotated as MraY), which initiates the lipid cycle of peptidoglycan cell wall biosynthesis by transfer of phospho-N-acetylmuramic acid-pentapeptide from UDP-N-acetylmuramic acid-pentapeptide to undecaprenyl phosphate, represents one such enzyme for which there are no marketed antibiotic drugs.3 Given the emergence and re-emergence of drug-resistance pathogens,4 the essential nature of peptidoglycan in the viability of bacteria, and the lack of a bacterial translocase I activity in mammals, MraY is an attractive target for the design and development of new antibiotics. Recent efforts in several laboratories have revealed that several structurally diverse natural products potently inhibit bacterial translocase I.3 This includes several families of nucleoside antibiotics that have unique chemical scaffolds relative to clinically used antibiotics. The liposidomycins belong to one such family of nucleoside antibiotics called fatty acyl nucleosides that also includes the caprazamycins isolated from Streptomyces sp. MK730-62F2.5 The liposidomycins, the structure of which was initially reported in 1988,6 are characterized by four moieties, a 5′-C-glycyluridine, a 2′-sulfated aminoribose, a diazepanone, and a β-hydroxy fatty acid moiety of variable carbon chain length modified with an unusual 3-methylglutaryl group at the β-position. Caprazamycins, in contrast to liposidomycins, contain an additional permethylated rhamnose and lack the 2′-O-sulfate moiety.5 During screening for new compounds that inhibit bacterial translocase I, we isolated a series of related compounds from Streptomyces sp. SANK 60405 termed A-90289s that had properties characteristic of the fatty acyl nucleoside antibiotics including variable fatty acid side chains. NMR analysis—including DQF COSY, HMBC, and NOESY experiments—and MS analysis with collision-induced dissociation (CID) were consistent with A-90289A, consisting of a β-hydroxy palmitic acid moiety and having a structure identical to that of caprazamycin A but also containing a sulfate group characteristic of liposidomycins B-I and the other liposidomycins (Scheme 1). However, in contrast to the reported structure of liposidomycin B-I, the CID MS spectrum was consistent with the sulfate group attached at the 2′-hydroxyl group as opposed to the 2′′-hydroxyl group (manuscript in preparation), and this might be the case for liposidomycins.6 Structure of model fatty acyl nucleoside antibiotics. The structure of A-90289 has features similar to the reported structures of caprazamycins and liposidomycin B-I. The biosynthetic gene cluster of caprazamycin was recently cloned and characterized.7 Heterologous expression in Streptomyces coelicolor M512 allowed for the prediction of 22 open reading frames (orfs) involved in caprazamycin biosynthesis, highlighted by three orfs encoding O-methyltransferases involved in the biosynthesis of the permethylated rhamnose moiety, an orf encoding a predicted glycosyltransferase (cpz31) expected to attach the rhamnose moiety to the aglycon, and an orf encoding a serine hydroxymethyl transferase (SHMT, cpz14) homologue with putative role in 5′-C-glycyluridine formation. The function of the acyltransferases that attach the fatty acid and 3-methylglutaryl moieties, Cpz23 and Cpz21, were subsequently confirmed by gene inactivation and isolation of the corresponding metabolites lacking the respective moiety.7 Studies were initiated to identify the A-90289 gene cluster from Streptomyces sp. SANK 60405 to allow a complete comparison of clusters and, importantly, to identify the mechanism of sulfate incorporation, which remains poorly explored for bacteria.8 Thus, the whole genome was sequenced by using 454 technology, and complete analysis of the resulting 1199 contiguous sequences encompassing 7.9 MB of unique DNA sequence revealed one contiguous sequence containing three tandem O-methyltransferases and several orfs encoding proteins of expected function. A close comparison of this contiguous sequence and the caprazamycin gene cluster revealed a nearly identical genetic architecture with homologous proteins having 70–92 % sequence identity, and we therefore annotated orfs in this contiguous sequence as lipA, lipB, etc., for liposidomycin-type biosynthetic gene cluster (Figure 1). While the caprazamycin gene cluster was proposed to have an upstream boundary at cpz9, encoding a putative transcription regulator, we identified an upstream gene (lipB) within the A-90289 locus that was predicted to encode an aryl sulfotransferase with sequence similarity to SACE_7046 from Saccharopolyspora erythraea, which is annotated as a hypothetical protein but has significant sequence similarity to known bacterial aryl sulfotransferase. This LipB protein presumably has an identical function to Cpz4, which was designated as a hypothetical protein but also reported to have sequence similarity to SACE_7046.7 Unfortunately, the sequence of cpz4, along with all orfs upstream of cpz9, was not available in the protein databanks for comparisons. However, examination of the proposed function of cpz3 to cpz8 and bioinformatics analysis of lipA to lipE within the A-90289 gene cluster suggests most orfs are conserved as depicted in Figure 1. Indeed, these same orfs have also been identified within the biosynthetic gene cluster of liposidomycin from Streptomyces griseosporeus SN-1061M.9 The only obvious differences are 1) an additional orf is found between lipA and lipB within the A-90289 locus that encode a putative pirin-like protein of unknown function, and 2) cpz7, which encodes a protein with similarity to adenosine-3′-phosphate-5′-phosphosulfate phosphatase, appears to be absent from the A-90289 locus. Depiction of the upstream boundary of the reported caprazamycin gene cluster and the gene cluster identified for A-90289 biosynthesis. The putative function of shared orfs is listed with letters representing orfs from the A-90289 cluster and the reported numbers representing the caprazamycin gene cluster. The sequence similarity between gene products that are available in the protein databank is given between the gene clusters. The three orfs targeted for inactivation are highlighted. SUT, sulfotransferase; SHMT, serine hydroxymethyltransferase; and GT, glycosyltransferase. To confirm the identity of the cluster, elucidate the role of lipB in A-90289 biosynthesis, and establish a system to produce new compounds by using combinatorial biosynthesis, a genetic system was developed within the A-90289 producing strain. A double-crossover strategy was employed to replace genes of interest with an ermE cassette conferring resistance to erythromycin (Figure 2 A).10 The lipK gene was initially targeted for replacement, and the generation of the mutant strain was confirmed by Southern blot analysis (Figure 2 B). As previously mentioned, the wild-type strain produces a series of related A-90289s that all vary within β-hydroxy fatty acid moiety, and control fermentations revealed a series of peaks with the expected masses of various acylated A-90289s (Figure 3 A). In contrast, replacement of the lipK gene encoding the putative SHMT with the ermE resistance cassette completely abolished production of all A-90289s. Unfortunately, the ΔlipK mutant strain was unable to be retransformed for complementation in trans, thus it was possible that the loss of production was due to polar effects. Therefore, lipB, representing—or near to—the upstream boundary, and lipB1, representing the downstream boundary, were targeted for replacement (see the Supporting Information) with the expectation that these mutants would produce new A-90289 metabolites, thus essentially eliminating the concern that loss of A-90289 production is due to a polar effect. Fermentation of the ΔlipB1 mutant strain and examination of the culture extract by HPLC revealed a series of peaks with earlier retention times that mirrored the peaks observed for the extract from the wild-type strain (Figure 3 A). LC-MS analysis of the new peaks identified these compounds as the respective A-90289s lacking the permethylated rhamnose moiety, thus successfully converting Streptomyces sp. SANK 60405 from an A-90289 producer into a liposidomycin-type producer. Similarly, fermentation of the ΔlipB mutant strain and examination of the culture extract by HPLC revealed a series of peaks that mirrored those observed from the culture broth of the wild-type strain, except in this case the peaks eluted at a later retention time than the corresponding peaks from the wild-type strain (Figure 3 A). LC-MS analysis of these peaks identified these compounds as the respective desulfo-A-90289s, including an observed [M−H]−1 ion of m/z 1145 consistent with the molecular formula for desulfo-A-90289A. Thus, Streptomyces sp. SANK 60405 was successfully converted into a caprazamycin producer, thereby establishing that LipB plays a critical role in sulfate incorporation. Generation and confirmation of mutant strains. A) The strategy utilized to generate gene replacements highlighted for the SHMT gene, lipK. Indicated are the locations of the BamHI sites within the wild-type DNA and the inserted ermE resistance gene. B) Genetic organization and restriction digest pattern for the expected mutant genotype. C) Genomic DNA was isolated from the wild-type strain (lane 1) and mutant strains (lanes 2 and 3) and digested with BamHI. Southern blot analysis with the selected probes yielded DNA fragments of the expected size when compared with the DNA marker (lane M) observed with ethidium bromide (EtBr). Analysis of the production of A-90289s from the wild-type and mutant strains. A) HPLC analysis of the extract from the wild-type strain revealed a series of related peaks with expected masses for A-90289s with variable β-hydroxy fatty acid moieties. Peaks are arbitrarily labeled for comparison purposes only, excluding peaks A and B, the structures of which are depicted in Scheme 1. The A-90289 derivative isolated from the mutant strain that lacks the expected moiety is labeled with the same letter, and the observed masses are included for comparisons. WT, wild-type extract; ΔGT, extract from the ΔlipB1 mutant strain; ΔSHMT, extract from the ΔlipK mutant strain; and ΔSUT, extract from the ΔlipB mutant strain. B) Antibacterial activity of extract from the wild-type and mutant strains. The sulfate-type liposidomycins were previously demonstrated to have no activity against Mycobacterium phlei IFO 3158; this is in contrast to the large zones of inhibition observed with nonsulfated liposidomycins.11 Therefore, the A-90289s and derivatives produced by genetic engineering were analyzed for their antibacterial activity against Bacillus subtilis ATCC 6633. As expected, no zone of inhibition was observed with sulfated A-90289s or derivatives produced from the ΔlipB1 mutant strain, while a clear zone of inhibition was observed for nonsulfated A-90289s (caprazamycins) generated from the ΔlipB mutant strain (Figure 3 B). Given that microorganisms often utilize covalent modification as a mechanism for self resistance to antibiotics,12 sulfate incorporation that is likely catalyzed by LipB might represent a self resistance strategy that leads to inactivation of A-90289s and lipsodiomycins. The putative sulfotransferase function of LipB was next explored in vitro. The gene was cloned and inserted into an E. coli expression vector, and the recombinant protein was purified by IMAC to reveal a dominant band of the expected size by SDS-PAGE (Figure 4 A). Desulfo-A-90289A was isolated and purified from the ΔlipB mutant strain (Figure 3), and incubations of the acceptor substrate with the sulfate donor p-nitrophenylsulfate revealed LipB readily converted the desulfo-compound to A-90289A, which was subsequently confirmed by MS analysis by using authentic A-90289A as a standard (Figure 4 B). This result established that LipB is indeed a sulfotransferase involved in A-90289 biosynthesis. In vitro characterization of LipB. A) SDS-PAGE analysis of purified His6-LipB (expected MW of 58.3 kD). Lane 1, MW standards and lane 2, purified His6-LipB. B–D) HPLC analysis of LipB activity at the indicated time points using p-nitrophenylsulfate as a sulfate donor and B) desulfo-A-90289, C) uridine, and D) 3′-deoxyuridine as sulfate acceptors. To explore the LipB reaction in more detail while circumventing the need to isolate large amounts of desulfo-A-90289A, surrogate acceptor substrates were tested. Interestingly, incubations of uridine with LipB and p-nitrophenylsulfate resulted in the formation of a new peak observed by HPLC (Figure 4 C). This new peak was purified and LC-MS analysis yielded an (M−H)− ion of m/z 323.1, consistent with the molecular formula for the monosulfated uridine (expected m/z 323.0). To obtain further data on acceptor specificity, mono-, di-, or triphosphate uridine nucleotides and the remaining canonical nucleosides and nucleotides were tested as substrates, but no new peaks were observed; this suggests the enzyme has some specificity with respect to the 5′-substituent and the uracil base. With uridine as an acceptor substrate, the sulfate donor specificity of LipB was also explored. Thus, LipB was incubated with 3′-phospoadenosine-5′-phosphosulfate (PAPS), the universal sulfate donor in eukaryotes for nonaryl alcohol acceptors; however, no reaction was observed. Finally, to probe the regiospecificity of sulfate incorporation, both 2′- and 3′-deoxyuridine were individually tested for activity with LipB. While no new peak was observed with 2′-deoxyuridine under conditions of excess enzyme, a new peak was observed with 3′-deoxyuridine as a substrate (Figure 4 D). This new peak was purified and LC-MS analysis yielded an (M−H)− ion of m/z 307.1, consistent with the molecular formula for the monosulfated 3′-deoxyuridine (expected m/z 307.0). Therefore, the results are consistent with sulfate transfer occurring at the 2′-hydroxyl, which substantiates the structural analysis of A-90289s that are consistent with a 2′-sulfate and not a 2′′-sulfate (Scheme 1). Sulfate incorporation in mammals is a key step in detoxification of aromatic hydrocarbons and for molecular recognition and signaling.8 Enzymes that catalyze this event have hitherto been identified as PAPS-dependent sulfotransferases and are represented by estrogen and heparin sulfotransferases.8 In bacteria, sulfate transfer enzymes are also known, but these enzymes, which form a distinct class of enzymes named aryl sulfotransferases, utilize a PAPS-independent mechanism that employ an aryl sulfate donor substrate and phenolic-type acceptor substrate.13–16 LipB has closest sequence homology to the latter class of enzymes, and we have demonstrated here that p-nitrophenylsulfate does serve as the sulfate donor as expected from sequence analysis. Interestingly, however, the acceptor substrate for LipB is not a phenolic-type compound but instead the 2′-hydroxyl of a uridine moiety; thus, this suggests a fundamental difference in catalysis by LipB and other characterized aryl sulfotransferases. Recent structural and biochemical analysis of an E. coli aryl sulfotransferase has revealed a disulfide bond is essential for function,13 but interestingly LipB does not contain any cysteine residues and therefore this structural variation might contribute to this fundamental difference. The biochemical ramification of this observation and the mechanism of LipB are currently under investigation. The biosynthetic genes for a given metabolite in Streptomyces are typically clustered within one region of the chromosomal DNA. The identification of LipB as a bona fide sulfotransferase and LipB1 as a glycosyltransferase suggests the biosynthetic gene cluster of A-90289 ranges from lipA–lipB1, consisting of minimally 28 genes involved in the biosynthesis, resistance, and regulation of A-90289s. This consists of an additional five orfs to what was initially described for the caprazamycin gene cluster7 that have also been identified within the liposidomycin gene cluster.9 Of these five orfs, an orf for a putative hydroxymethylglutaryl-CoA synthase (cpz5) and a type III polyketide synthase (cpz6) were suggested to be outside of the caprazamycin gene cluster, yet the biosynthesis of the 3-methylglutaryl moiety could not be rationalized from the remaining genes within the cluster or from the genome of the heterologous host, of which the entire genome sequence is known.7 The accumulated data now suggests these two orfs might indeed be involved in A-90289 biosynthesis, potentially in the assembly of the 3-methylglutaryl moiety. Alternatively, and perhaps a more intriguing possibility that would be consistent with the heterologous production experiments with caprazamycin, is that cpz5, cpz6, and potentially the remaining orfs, are involved in the biosynthesis of an aryl sulfate compound that serves as the in vivo sulfate donor for LipB. Thus, orfs upstream of orf9 would not be expected to contribute to caprazamycin biosynthesis as previously demonstrated, but are essential for sulfate incorporation during A-90289 and liposidomycin biosynthesis. Interestingly, new sulfated caprazamycins have now been isolated, and it has been demonstrated that these upstream orfs are indeed required for sulfate incorporation.9 Identification of the A-90289 gene cluster and establishment of a genetic system will now facilitate studies geared toward further understanding the role of these and other orfs involved in the biosynthesis of fatty acyl nucleoside antibiotics. Chemicals: Uridine, 2′-deoxyuridine, 3′-deoxyuridine, and p-nitrophenylsulfate were purchased from Sigma–Aldrich. Production and detection of A-90289: Streptomyces sp. SANK 60405 was grown on DifcoTM ISP medium 2 (Becton, Dickinson and company, Sparks, MD, USA) to prepare spore suspensions. An aliquot of spore suspension was added to a test tube containing T-12B medium (5 mL total; 0.3 % glucose, 0.3 % Soybean meal, 0.03 % Difco yeast extract, 0.04 % CaCO3, 0.02 % MgSO4⋅7H2O, 0.005 % antiform CB-442, pH7.2). The culture was incubated with shaking (310 rpm) for three days at 28 °C. The resulting culture broth (1 mL) was transferred into an Erlenmeyer flask (100 mL) containing T-12B (20 mL) and cultivated for seven days with shaking (210 rpm) at 28 °C. Genetically modified mutants were cultured identically except lincomycin A (10 μg mL−1) was added. Whole culture broth was extracted with an equal volume of acetone and centrifuged to remove cell debris, and the extract was applied for HPLC analysis. A-90289s were detected using a C-18 reversed-phase HPLC with an analytical Unison UK-C18 column (75 mm×4.6 mm, Imtakt Corporation, Kyoto, Japan). A series of linear gradients was developed from ammonium formate (10 mm; pH 3.5) in acetonitrile (10 %; A) to acetonitrile (90 %; B) in the following manner (beginning time and ending time with linear increase to % B): 0–2 min, 25 % B; 2–12 min, 75 % B; 12–15 min, 75 % B. The flow rate was kept constant at 1.0 mL min−1, and elution was monitored at 260 nm by using an Agilent 1100 Series Quaternary LC System (Agilent Technologies, Inc. Santa Clara, CA, USA). Electrospray ionization-MS was conducted using an Agilent 1100 HPLC-MSD instrument (Agilent Technologies, Inc. Santa Clara, CA, USA). Desulfo-A-90289A was extracted from the culture broth of the ΔlipK mutant strain using acetone followed by purification using an HP-20 column. The fraction containing desulfo-A-90289A, which eluted with acetone (70 %), was further purified by C18 reversed-phase HPLC. Genome DNA sequencing, gene prediction and annotation: The isolated genomic DNA from Streptomyces sp. SANK 60405 was sequenced by Takara Bio Inc. (Shiga, Japan) with a Roche GS FLX system. Putative protein-coding sequences were predicted using glimmer2 and CRITICA trained with all annotated open reading frames (orfs) of S. coelicolor A3(2) (Accession no. NC_003888.3), plasmids SCP1 (Accession no. NC_003903.1) and SCP2 (Accession no. NC_003904.1) and then manually confirmed using the BLAST search tools from the National Center for Biotechnology Information (Bethesda, MD, USA). Genomic library construction: Genomic library of Streptomyces sp. SANK 60405 was constructed according to our previous report.17 Approximately 20 000 colonies from the genomic library were screened by colony hybridization by using a digoxigenin-labeled lipK gene encoding the putative SHMT. Six representative positive clones, Lip01–06, were selected for completion of the A-90289 cluster sequence using a Roche GS FLX system (Takara Bio Inc.). The DNA sequence has been deposited in DDBJ under the accession number AB530986. Construction of plasmids for gene inactivation: To inactivate lipK, a 1.2 kb DNA fragment downstream of lipK and a 1.5 kb DNA fragment upstream of lipK were amplified using the following primer pairs: 5′-GCG AGA TCT GGT CCG CGA GC-3′/5′-GCG GCA TGC GAG GGG CCC TGT GTC CG-3′ and 5′-GCG CAT ATG TCA TCA CCT CAA TGC CG-3′/5′-GCG ACG CGT CCG CCC GAT G-3′, respectively. After sequencing to confirm PCR fidelity, resulting DNA fragments were ligated to the 1.8 kb DNA SphI-NdeI fragment that contains the erythromycin resistance gene, ermE,9 a gift from Prof. Dr. Ben Shen, University of Wisconsin at Madison. The obtained 4.5 kb DNA fragment containing ermE was introduced into pO J260,9 also a gift from Prof. Dr. Ben Shen, resulting pO J260ΔlipK. The plasmid pO J260ΔlipK was introduced into Streptomyces sp. SANK 60405 by PEG-mediated protoplast transformation.9 Colonies that were apramycin sensitive and lincomycin resistance were identified as the ΔlipK mutant strains, and the genotype was confirmed by Southern blot analysis (Figure 3 B). To inactivate lipB and lipB1, an identical strategy was used to generate pO J260ΔlipB and pO J260ΔlipB1. A 1.8 kb DNA fragment downstream of lipB and 1.55-kb DNA fragment upstream of lipB were amplified using the following primer pairs: 5′-GCG TCT AGA GGA TCG TCA CGG CGG GTC-3′/5′-GCG ACG CGT CGG CCT CCG CCG AGC TGC-3′ and 5′-GCG ACT AGT TTG TGG CCG GCC ATC ACC G-3′/5′-GCG AGA TCT GTC AGG GCG GCC AGC TCC-3′, respectively. A 1.7 kb DNA fragment downstream of lipB1 and a 1.6 kb DNA fragment upstream of lipB1 were amplified using the following primer pairs: 5′-GCG ACT AGT GTG GCG AGG ACG TGA TGC-3′/5′-GCG CAT ATG CGC GAA CGC CGT CAC CCG-3′ and 5′-GCG GCA TGC GCA TGG CTC ACC TCG TTC G-3′/5′-GCG AGA TCT GAT CCT CGA CGG CTT CGG-3′, respectively. After antibiotic selection, Southern blot analysis confirmed the expected genotype (Supporting Information). Bioassay of A-90289 and its derivatives: Antimicrobial activity was conducted using Bacillus subtilis ATCC 6633 as an indicator strain. 30 μL of acetone-extracted culture broth was applied to a paper disc on pearl-core nutrient broth (Eiken Chemical Co., Ltd, Tokyo, Japan) that included a B. subtilis spore suspension (0.02 %). The assay plate was incubated at 37 °C for 24 h. Cloning and expression of lipB: The gene for lipB was amplified by PCR using Expand Long Template PCR System from Roche (Indianapolis, IN, USA) with supplied Buffer 2, dNTPs (200 mM), DMSO (5 %), pN1 (10 ng), DNA polymerase (5 U), and 10 mM each of the following primer pair: (forward) 5′-GGT ATT GAG GGT CGC ATG GTC CGG ACG AGA ACG AC-3′/(reverse) 5′-AGA GGA GAG TTA GAG CCT CAG CGC ACC CGC GCG G-3′. The PCR program included an initial hold at 94 °C for 2 min, followed by 30 cycles of 94 °C for 10 s, 56 °C for 15 s, and 68 °C for 90 s. The gel-purified PCR product was inserted into pET-30 Xa/LIC by using ligation-independent cloning as described by Novagen (Madison, WI, USA) to yield pET30-lipB, which was subsequently sequenced at the University of Kentucky Advanced Genetic Technologies Center to confirm PCR fidelity. Preparation and activity of LipB: E. coli BL21(DE3)/pET30-lipB cells were lysed using a French Press with 15 000 psi, and the protein purified using a Profinia protein purification system equipped with a Bio-Scale Mini Profinity IMAC cartridge (1 mL) and a Bio-Scale Mini Bio-Gel® P-6 desalting cartridge (10 mL; Bio-Rad Laboratories, Hercules, CA, USA). The purified protein was concentrated using an Amicon Ultra 10 000 MWCO centrifugal filter (Millipore) and stored as glycerol stocks (40 %) at −20 °C. The assay mixture with desulfo-A-90289A consisted of Tris-HCl (50 mM, pH 8.0), p-nitrophenylsulfate (2 mM), purified desulfo-A-90289A , and LipB (0.6 mg mL−1) at 30 °C. The reaction was monitored as described for analysis of A-90289 production except an isocratic mobile phase of ammonium formate (10 mM), formic acid (0.1 %), and acetonitrile (55 %) was used. The assay mixture with uridine consisted of potassium phosphate (50 mM, pH 8.0), uridine (1.5 mM) or related substrate, p-nitrophenylsulfate (2 mM), and LipB (0.9 mg mL−1) at 30 °C. The reaction was monitored by using a C-18 reversed-phase HPLC with an analytical Apollo C-18 column (250 mm × 4.6 mm, 5 μm). A series of linear gradients was developed from TFA (0.1 %) in acetonitrile (2.5 %; A) to acetonitrile (90 %; B) in the following manner (beginning time and ending time with linear increase to % B): 0–8 min, 100 % B; 8–18 min, 60 % B; 18–25 min, 95 % B; 25–32 min, 95 % B; and 32–35 min, 0 % B. The flow rate was kept constant at 1.0 mL min−1, and elution was monitored at 260 nm. Peaks were collected, dried, and analyzed by electrospray ionization-MS using an Agilent 6120 Quadrupole MSD mass spectrometer (Agilent Technologies, Inc. Santa Clara, CA, USA) equipped with an Agilent 1200 Series Quaternary LC System and an Eclipse XDB-C18 column (5 μm, 4.6×250 mm, 80 Å). This work is supported in part by the Kentucky Science and Technology Foundation (S.V.L.). Detailed facts of importance to specialist readers are published as ”Supporting Information”. Such documents are peer-reviewed, but not copy-edited or typeset. They are made available as submitted by the authors. Please note: The publisher is not responsible for the content or functionality of any supporting information supplied by the authors. Any queries (other than missing content) should be directed to the corresponding author for the article.
MoreTranslated text
Key words
antibiotics,bacterial translocase I,biosynthesis,nucleosides,sulfotransferase
AI Read Science
Must-Reading Tree
Example
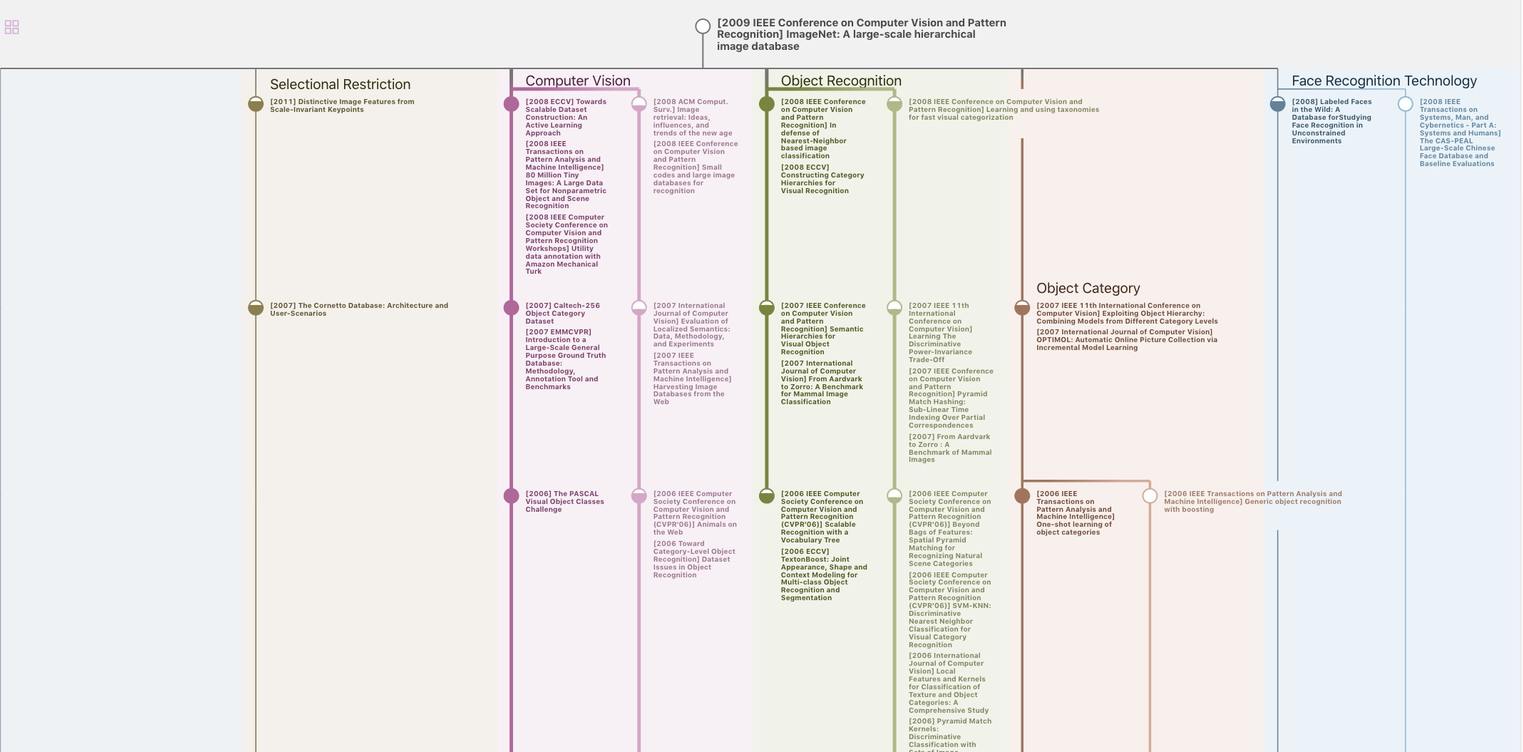
Generate MRT to find the research sequence of this paper
Chat Paper
Summary is being generated by the instructions you defined