Nanocrystallization-induced large room-temperature compressive plastic strain of Ti40Zr25Ni8Cu9Be18 BMG
Journal of Alloys and Compounds(2011)
摘要
Research highlights ▶ Combination of neutron diffraction, high-resolution transmission electron microscopy and thermal analysis measurements. ▶ Determination of the microstructure of as-cast Ti 40 Zr 25 Ni 8 Cu 9 Be 18 BMG. ▶ (Ti,Zr)Be 2 nanocrystals with the average size of 4 nm homogeneously distributed into the amorphous matrix. ▶ large room-temperature compressive plastic strain, up to 8.0% at a constant strain rate of 5 × 10 −4 S −1 . Abstract By combining neutron diffraction, high-resolution transmission electron microscopy and thermal analysis measurements, the microstructure of as-cast Ti 40 Zr 25 Ni 8 Cu 9 Be 18 BMG with diameter of 2 mm was identified to be quenched-in nuclei/amorphous matrix. The quenched-in nuclei transform to (Ti,Zr)Be 2 nanocrystals with the average size of 4 nm homogeneously distributed into the amorphous matrix during deformation, which could be responsible for the large room-temperature compressive plastic strain, up to 8.0% at a constant strain rate of 5 × 10 −4 s −1 . Keywords Bulk metallic glass Titanium alloy Microstructure Quenched-in nuclei Nanocrystallization Plastic strain 1 Introduction Ti-based bulk metallic glasses (BMGs) have attracted widespread attention for their scientific importance and potential applications as lightweight structural materials [1–10] . Ti-rich Ti–Zr–Ni–Cu–Be alloys are typical Ti-based BMGs, generally have good combination of GFA and mechanical properties, especially large room-temperature compressive plastic strain [5–10] ; however, the reason why Ti–Zr–Ni–Cu–Be BMGs exhibit large compressive plastic strain is not clear, and still in argument. Park et al. [8] found that the Ti-rich Ti–Zr–Ni–Cu–Be BMGs whose first crystallization product was quasicrystalline phase generally exhibited relatively large plastic strain, and attributed the enhancement of plastic strain to the existence of quenched-in icosahedral nuclei embedded in the amorphous matrix. While recently, Fornell et al. [10] argued that the intrinsic plasticity of Ti–Zr–Ni–Cu–Be BMGs was due to its elastic properties, namely, the relatively good plasticity was related to rather low ratio of elastic shear modulus ( μ ) to bulk modulus ( B ), μ / B . In addition, Ohkubo et al. [9] argued that the plasticity of Ti 40 Zr 25 Cu 12 Ni 3 Be 20 alloy does not originate from the glassy phase itself but is closely related to the amount of nanocrystals embedded in the metallic glass matrix. The mechanical response for a material is correlated with its microstructure. In addition, for metallic glasses, since their characteristic of thermodynamic metastability, the structural changes during deformation caused by strain energy should also be considered during the analysis of deformation behavior. So, it is possible to construct the correlation between the large room-temperature plastic strain and the structural instability of metallic glasses. In this study, we present the large room-temperature compressive plastic strain (up to 8.0% at a strain rate of 5 × 10 −4 s −1 ) of Ti 40 Zr 25 Ni 8 Cu 9 Be 18 BMG, and a discussion based on the analysis of structural nature of the as-cast sample and its structural changes during deformation. The nanocrystallization of the quenched-in nuclei in the amorphous matrix during deformation should be responsible for the enhancement of plastic strain. 2 Experiment The ingots of master alloy with nominal composition of Ti 40 Zr 25 Ni 8 Cu 9 Be 18 (at.%) were prepared by melting elements of 99.8–99.999 wt.% purity with high frequency electromagnetic induction in a water-cooled copper crucible under a high purity argon atmosphere. The ingot alloys were remelted at high temperature several times to ensure the compositional homogeneity. Cylindrical specimens with 2 mm in diameter and 40 mm in length were prepared by injection casting into a water-cooled copper mould via an electromagnetic levitation-melt state under a high purity argon atmosphere before casting to obtain a completely melted state. Alloy's oxygen content was measured <400 ppm. DSC parameters measured at 10 K/min are: Δ T = 53 K between T g = 575 K and first crystallization peak T x 1 = 628 K [11] . The glassy state of alloy was examined by high-resolution transmission electron microscopy (HRTEM, JEM-3010). Isothermal crystallization was studied using differential scanning calorimetry (DSC, Netzsch DSC 404 s) in a flowing argon atmosphere. Uniaxial compression tests were performed on cylindrical rods of 2 mm in diameter and 3.5 mm in length by using a MTS 4 M testing machine at room-temperature with constant strain rate of 5 × 10 −4 s −1 . The sample end surfaces were polished to be parallel to each other and perpendicular to the loading axis. BN powder was used as lubricant to reduce friction between testing samples and the platen of the machine. The fractured samples were observed in a JEOL-JSM6400 scanning electron microscope (SEM) and TEM. 3 Results 3.1 Microstructure identification of as-cast Ti 40 Zr 25 Ni 8 Cu 9 Be 18 BMG Fig. 1 shows a bright-field TEM image with inset of selected-area electron diffraction pattern (SADP) from the as-cast Ti 40 Zr 25 Ni 8 Cu 9 Be 18 alloy, reveals a uniform, featureless homogeneous contrast, lacking any long-range topological order, no regularity of the lattice fringes and a series of diffuse halo rings, respectively, indicating a monolithic amorphous phase. Here, we recall the results obtained in the in-situ neutron diffraction investigation performed on the as-cast Ti 40 Zr 25 Ni 8 Cu 9 Be 18 alloy which has been presented in Ref. [11] using λ = 2.534 Å. At the beginning of the experiment, the characteristic diffraction pattern of an amorphous compound with short range order (bump maximum at 2 θ = 68° d = 2.257 Å) is observed. At 610 K (608 K in DSC experiment), some new peaks appear at 2 θ = 39.34° and 65.54° corresponding to d spacings 3.749 and 2.332 Å, respectively, these peaks are diffuse at the beginning and correspond certainly to very tiny crystals, then sharper with time and temperature increasing. With only 2 peaks it is difficult to ascertain a phase, but we can exclude some hypothesis, in particular α- or β-Ti, which did not present a peak at d = 3.749 Å. In the same way the icosahedral phase often observed in phases with beryllium presents a double peak around d = 2.34 Å. In simple binary phases, only the TiBe 2 (fcc, a = 6.45 Å) phase presents 2 peaks close to the observed peaks, by refining the cell parameter, the value a = 6.603 Å has been determined. It could be explained by a partial replacement of Ti by a bigger atom such as zirconium. However the ZrBe 2 compound is hexagonal, so only partial replacement must be assumed. The formation of the (Ti,Zr)Be 2 phase as primary crystallization phase (PCP) is in agreement with previous primary crystallization studies of BMG containing beryllium phase. We have observed, during the crystallization of Zr 46.75 Ti 8.25 Cu 7.5 Ni 10 Be 27.5 phase, the formation of ZrBe 2 and a quasicrystalline phase [12] as PCP and whose TEM images are very similar in shape and size. Depending on the history of the glass formation (sample diameter, cooling technique, oxygen presence), different phases can be formed because of the different atomic structures (short range order) possible in the glass state. It is why the observations of Kim et al. [7] of a quasicrystalline phase as PCP may be also the result of the BMG synthesis process, different from our samples. More information about the in-situ neutron diffraction study of Ti 40 Zr 25 Ni 8 Cu 9 Be 18 alloy upon heating has been presented in Ref. [11] . However, the isothermal DSC curve (at 340 °C, in the glass transition region, see Fig. 2 ) shows a monotonically decreasing calorimetric signal, which is the evidence that the crystallization process involves only the grain coarsening or growth of quenched-in nuclei. For grain coarsening or growth of quenched-in nuclei, the kinetics of the two processes is fundamentally different although they may exhibit similar calorimetric behavior. Growth of quenched-in nuclei in metallic glasses involves a solid state transformation from an amorphous state to a crystalline state, while grain coarsening occurs in micro-crystalline or nanocrystalline materials just corresponding to the reduction in interface enthalpy due to the annihilation of grain boundaries. Obviously, for Ti 40 Zr 25 Ni 8 Cu 9 Be 18 BMG, no crystals can be detected by neutron diffraction and HRTEM measurements, confirming the growth of quenched-in nuclei as the origins for the decreasing calorimetric signal. Actually, we have performed non-isothermal DSC to investigate the crystallization mechanism (see Ref. [13] ), and the calculated Avrami exponent n for the first crystallization event falls in the range of 1.4–1.6, implying a diffusion-controlled three-dimensional growth of pre-existing nuclei for the first crystallization event, as also confirms the quenched-in nuclei/amorphous matrix structure of the as-cast Ti 40 Zr 25 Ni 8 Cu 9 Be 18 BMG. 3.2 Room-temperature compressive properties of as-cast Ti 40 Zr 25 Ni 8 Cu 9 Be 18 BMG Fig. 3 shows a typical room-temperature uniaxial compressive true strain–stress ( σ – ɛ ) curve of as-cast Ti 40 Zr 25 Ni 8 Cu 9 Be 18 BMG deformed at a constant strain rate of 5 × 10 −4 s −1 . It is found that it shows a large elastic strain of 2.1%, and had a maximum strength of 1850 MPa and a considerable plastic strain of 8.0%. In addition, after yielding, obvious serrated flow can be observed (see the enlarged image in Fig. 3 ). The occurrence of inhomogeneous plastic flow indicates the initiation and propagation of individual shear bands during quasistatic loading [14] . And in these shear bands there is a sudden decrease of viscosity [15] , which brings about load drops and a concomitant mechanical softening. Fig. 4 shows the outer surfaces of the fractured Ti 40 Zr 25 Ni 8 Cu 9 Be 18 sample. Surprisingly, the multiple shear bands including primary shear bands and branched secondary shear bands (see Fig. 4 (b)) are distributed homogeneously over the whole surface, explaining the large plastic strain observed during compressive deformation. It is well known that for common BMGs, one major shear band dominates the deformation and results in a sudden fracture, in this study we found multiple shear bands on the outer surface of fractured Ti 40 Zr 25 Ni 8 Cu 9 Be 18 BMG, suggesting that a large number of shear bands simultaneously operate during the plastic deformation, the reason will be discussed in the following section. 4 Discussion The dissimilar intrinsic plasticity or brittleness in different BMG systems is essentially due to their different nature, e.g. (i) properties of constituent elements and composition, (ii) and structural configurations. It was reported that the high metalloid content as well as the presence in major quantities of a metallic constituent that has a body centered cubic structure in its crystalline state (e.g. Mg-based metallic glasses), leads to intrinsic brittleness [16] . Spaepen [15] proposed that shear bands are initiated as a result of coalescence of the free volume, which was later confirmed by HRTEM [17] . Sung et al. [18] argued that alloys with a low melting temperature usually exhibit large plastic strain. In addition, the elastic moduli are apparently important parameters as a chain to understand the correlation between the structural characteristics and relevant mechanical properties. Lewandowski et al. [19] pointed out that the intrinsic plasticity or brittleness of metallic glasses is correlated with their Poisson's ratio, ν . The larger the ν is, the more ductile the metallic glasses become. Metallic glasses with a high Poisson's ratio ν > 0.31–0.32 or, equivalently, a ratio of elastic shear modulus ( μ ) to bulk modulus ( B ), μ / B , less than 0.41–0.43, are ductile. The value of μ / B for Ti 40 Zr 25 Ni 8 Cu 9 Be 18 metallic glass is measured to be 0.314, which is higher than that for Pd-based and Pt-based metallic glasses ( μ / B > 0.18) however lower than that for some more brittle metallic glasses ( μ / B > 0.43 for Mg-based and Ce-based metallic glasses) and oxide glasses ( μ / B > 0.7) [10] , indicating its moderate plasticity. Park et al. [8] found that the Ti-rich Ti–Zr–Ni–Cu–Be BMGs whose first crystallization product was quasicrystalline phase generally exhibited relatively large plastic strain, and attributed the enhancement of plastic strain to the existence of quenched-in icosahedral nuclei embedded in the amorphous matrix. However, for Ti 40 Zr 25 Ni 8 Cu 9 Be 18 BMG, the first crystallization product is not a quasicrystalline phase but (Ti,Zr)Be 2 phase, implying that the large plastic strain should be independent on the properties of primary phase, there must be other reason being responsible for the large compressive plastic strain of Ti 40 Zr 25 Ni 8 Cu 9 Be 18 BMG. The TEM image for the fractured Ti 40 Zr 25 Ni 8 Cu 9 Be 18 BMG under the condition of room-temperature compression at a constant strain rate of 5 × 10 −4 s −1 is shown in Fig. 5 . It can be seen that nanocrystals with an average size of 4 nm are homogeneously embedded in the amorphous matrix, demonstrating the occurrence of the transformation from quenched-in nuclei to nanocrystals during deformation. The formation of the nanocrystals may be due to the supply of the mechanical energy during compression, which is associated with its low thermal stability (activation energy for its first crystallization event is 235.2 kJ/mol [13] ) [20] , implying that the large plastic strain may be dependent on the thermal instability upon heating. It is well known that the nanocrystals may significantly affect the mechanical behavior of metallic glasses through the interaction with shear bands. Such nanocrystals become inhomogeneous energy barriers, and a propagating shear band might be forced to be deflected or branched when it reaches the high energy barrier region. This would result in the initiation of new shear bands. The increase of the total area of shear bands due to the formation of multiple shear bands and deflection in the individual shear bands lead to appreciable macroscopic plasticity at low strain rate. For example, it was reported recently that the as-cast Cu 50 Zr 50 BMGs exhibited a compressive plastic strain of more than 50% at room-temperature due to a dispersion of embedded nanocrystals, which was explained by the suppression of shear softening through nanocrystal coalescence [21] . Based on the analysis above, the large compressive plastic strain of Ti 40 Zr 25 Ni 8 Cu 9 Be 18 BMG may result from its high value of Poisson's ratio (or low value of the ratio of elastic shear modulus ( μ ) to bulk modulus ( B )) as well as the homogeneous nanocrystallization of the quenched-in nuclei during deformation due to its low thermal stability which can disperse the strain localization upon compressive loading, resulting in the generation of multiple shear bands. 5 Conclusions The microstructure of as-cast Ti 40 Zr 25 Ni 8 Cu 9 Be 18 BMG was identified for the first time by combining neutron diffraction, high-resolution transmission electron microscopy and thermal analysis measurements, and the room-temperature compression test was also performed on this alloy. It is found the existence of quenched-in nuclei in the amorphous matrix for the as-cast sample. The primary crystallization phase is not I-phase as previously reported, but (Ti,Zr)Be 2 phase, which may result from the different history of the glass formation. The large room-temperature compressive plastic strain up to 8.0% at a constant strain rate of 5 × 10 −4 s −1 could be mainly attributed to the nanocrystallization of the quenched-in nuclei during deformation which is correlated with the thermal instability of the quenched-in nuclei in addition to its high value of Poisson's ratio (or low value of the ratio of elastic shear modulus ( μ ) to bulk modulus ( B )). Acknowledgements This work was supported by National Natural Science Foundation of China ( 50601011 ), National Basic Research Program of China ( 2007CB607603 ), Fundamental Research Project of National Defense of China ( A2720060295 ) and China Scholarship Council . We are very grateful to Q. Wang for helpful advice. References [1] G. Duan A. Wiest M.L. Lind A. Kahl W.L. Johnson Scr. Mater. 58 2008 465 468 [2] C.L. Ma S. Ishihara H. Soejima N. Nishiyama A. Inoue Trans. JIM 45 2004 1802 1806 [3] C.L. Ma H. Soejima S. Ishihara K. Amiya N. Nishiyama A. Inoue Mater. Trans. JIM 45 2004 3223 3227 [4] K.B. Kim X.F. Zhang S. Yi M.H. Lee J. Das J. Eckert Philos. Mag. Lett. 88 2008 75 81 [5] F.Q. Guo H.J. Wang S.J. Poon G.J. Shiflet Appl. Phys. Lett. 86 2005 091907 [6] Y.C. Kim J.H. Na J.M. Park D.H. Kim Y.H. Lee W.T. Kim Appl. Phys. Lett. 83 2003 3093 3095 [7] Y.C. Kim W.T. Kim D.H. Kim Mater. Sci. Eng. A 375–377 2004 127 135 [8] J.M. Park H.J. Chang K.H. Han W.T. Kim D.H. Kim Scr. Mater. 53 2005 1 6 [9] T. Ohkubo D. Nagahama T. Mukai K. Hono J. Mater. Res. 22 2007 1406 1413 [10] J. Fornell A. Concustell S. Suriñach W.H. Li N. Cuadrado Int. J. Plast. 25 2009 1540 1559 [11] J.L. Soubeyroux J.N. Mei J. Alloys Compd. 504S 2010 S239 S242 [12] B. Van De Moortèle T. Epicier J.L. Soubeyroux J.M. Pelletier Philos. Mag. Lett. 84 2004 245 256 [13] J.N. Mei, thesis, Joseph Fourier University & Northwestern Polytechnical University, 2009. [14] C.A. Schuh T.C. Hufnagel U. Ramamurty Acta Mater. 55 2007 4067 4109 [15] F. Spaepen Acta Mater. 23 1977 407 415 [16] U. Ramamurty M.L. Lee J. Basu Y. Li Scr. Mater. 47 2002 107 111 [17] J. Li Z.L. Wang T.C. Hufnagel Phys. Rev. B 65 2002 144201 [18] D.S. Sung O.J. Kwon E. Fleury K.B. Kim J.C. Lee D.H. Kim Y.C. Kim Met. Mater. Int. 10 2004 575 579 [19] J.J. Lewandowski W.H. Wang A.L. Greer Philos. Mag. Lett. 85 2005 77 87 [20] S.W. Lee M.Y. Huh E. Fleury J.C. Lee Acta Mater. 54 2006 349 355 [21] A. Inoue W. Zhang T. Tsurui A.R. Yavari A.L. Greer Philos. Mag. Lett. 85 2005 221 237
更多查看译文
关键词
Bulk metallic glass,Titanium alloy,Microstructure,Quenched-in nuclei,Nanocrystallization,Plastic strain
AI 理解论文
溯源树
样例
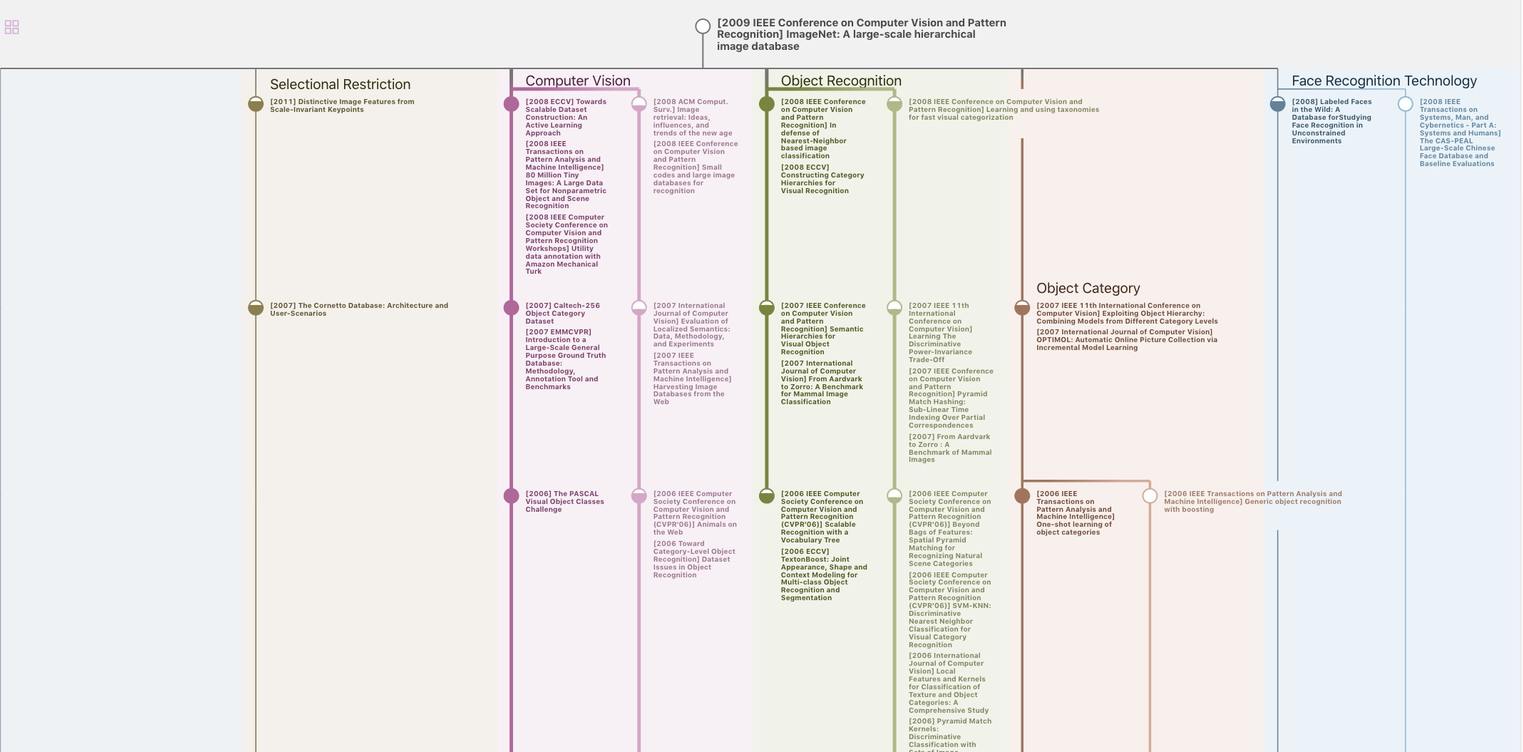
生成溯源树,研究论文发展脉络
Chat Paper
正在生成论文摘要