Superconducting RFQs
Physica C: Superconductivity and its applications(2006)
Abstract
At INFN-Legnaro the heavy ion injector PIAVE, based on two superconducting RFQ and eight quarter wave resonators (QWR), is at an advanced stage of beam commissioning. The RFQs (SRFQ1 and SRFQ2), built in full Nb within a stiffening Ti jacket, are 0.8 m in diameter and 1.4 and 0.8 m long respectively, with a resonant frequency of 80 MHz. The PIAVE beam is bunched by a normal conducting 3-harmonic buncher upstream the SRFQs. The SRFQs are specified to work at a peak surface field of 25.5 MV/m, a value which was exceeded in the test phase and has been recently confirmed in on-line tests. Phase and amplitude locking, versus both microphonics and pressure variations of the liquid helium bath, is the main issue. Since November 2004, the two SRFQs have been used quite extensively, for beam acceleration tests in PIAVE, showing a high degree of reliability. A 16 O 3+ pilot beam, received from an ECR ion source located on a high voltage platform, was used in the tests. The typical ion beam current was a few hundreds nA, even though it could be raised up to a few μA without any inconveniences. Beam tests with 132 Xe 18+ were made too. The paper reports the more recent results of on-line SRFQ tests and beam operation. Keywords RFQ Heavy ion linac Commissioning Superconducting RF 1 Introduction The tandem-ALPI heavy ion accelerator complex has been completed with a positive ion injector (PIAVE [1] ), featuring as accelerating structures two SRFQs and eight QWRs [2] , all in full Nb. In contrast to the XTU-tandem, PIAVE is able to feed the booster ALPI also with heavy ion species (up to U), delivered by an ECR ion source. Since November 2004, beam tests were carried out first of all through the SRFQs and later through the entire injector. A beam of 16 O 3+ , from the ECR ion source on a high voltage platform, was used for the tests (a current of ∼1–3 mA was typically available from the source). As expected, the energy output of PIAVE was 20.8 MeV. The measured transverse emittance was 0.1–0.15 mm mrad, to be compared to an expected value of 0.1 mm mrad. The recorded longitudinal emittance is still a factor 4 higher than the theoretical value (2 vs. 0.5 keV ns/A), but we suspect that instrumental errors still bear a non-negligible contribution, which is being eliminated. Beam transmission, between 85% and 100% in the cavity-free regions, turns out to be as high as 68% in the 3H-buncher [2] -to-SRFQ section (expected value 70%). Fig. 1 shows a photo of the compact PIAVE injector. The present paper is dedicated to the operation of SRFQ elements, core of the PIAVE injector, being their design construction and laboratory tests described in Refs. [3–6] . SRFQ1 and SRFQ2 are the first superconducting RFQ structures ever built for being put in regular beam operation. Their construction and operation went through many technological challenges, which were overcome in the test phase, such as end-plate RF joints [5] , gaseous He removal by the hollow electrodes [7] and others. The last critical issues could be focused only after assembling the resonators in the on-line cryostat: (1) To reach the specified accelerating field at the reference power dissipation ( P d ∼ 10 W), in the on-line cryostat. (2) To keep the SRFQs frequencies locked to the master oscillator, by compensating • the changes of the cavity volume due to drifts of the liquid He pressure (phenomenon with a time scale of seconds), • environmental vibrations, inducing oscillations of the SRFQ resonant frequency in a ms time scale. Locking tests were extensively performed during off-line tests [5] , but the real operational conditions could be faced only after assembling the resonators in the line cryostat and connecting it to the actual refrigeration system. (3) To find an accurate and fast method for setting the relative phase between the SRFQs and the phases of the bunching structures. (4) To check the SRFQ beam alignment, in cold conditions, with respect to the beam line and to verify the effect of misalignment on beam transport. 2 Q -Curves: reaching the SRFQS specifications The SRFQs main parameters are listed in Table 1 . At the maximum peak surface field of 25.5 MV/m (the design value, which was overcome since the off-line tests), the inter-electrode voltages of SRFQ1 and SRFQ2 are 148 and 280 kV respectively, values which are significantly higher than those achieved by typical normal conducting RFQs. The stored energy of the SRFQs is acceptably low for reasonable SEL phase-amplitude stabilization circuits [8] . Being the RFQ mostly a focusing structure, with a small on-axis component of the electric field, the ratio E a / E s,p is particularly low, with respect to other s.c. cavity types, i.e. 1 10 and 1 7.33 for the two resonators, respectively. Hence the design values of the accelerating field are limited to 2.4 and 3.4 MV/m for SRFQ1 and SRFQ2, respectively. In Fig. 2 , the Q vs. E a curves for the two SRFQ structures are shown. In order to reach these performances, each SRFQ underwent bake-out, at ∼350 K, for about 30 h. Keeping the intermediate shields at 77 K, resonant field emission (RFE) was processed in 24 h, while residual RFE low level processing (up to E a = 0.32 MV/m for SRFQ1 and E a = 0.85 MV/m for SRFQ2) required a few more hours. In addition, both cavities have always shown a very last RFE level at E a ∼ 0.9–1.2 MV/m: to overcome this level and to He-process non resonant field emission (FE) 2 h and 9 h for SRFQ1 and SRFQ2 were respectively required. The on-line Q -curves are lowered by the presence of VCX fast tuners [9] , which provide frequency control, with respect to the 80 MHz master oscillator, in a window of ±40 Hz (SRFQ1) and ±100 Hz (SRFQ2). The VCX fast tuners dissipate their power in a liquid nitrogen bath. The liquid He consumption was measured at 4 K, while the cryogenic system was set in the He-to-recovery configuration, confirming the Q -values of the old off-line curves. 3 On-line phase and amplitude stability In SRFQs, phase and amplitude locking is strongly linked to the pressure change rate of the liquid He bath. The resonators are equipped with slow mechanical tuners and VCX fast tuners. The liquid He pressure change, which has a time scale of seconds, induces frequency shifts which must be counteracted by the mechanical tuners. Each cavity features two tuners, one per end-plate: one is used to increase the frequency, the other to decrease it. The overall tuning range is ±100 kHz. When any of the tuners reaches the range limit, the direction of motion of both is reversed. The mechanical tuners are able to keep up with frequency changes of the resonator smaller than 2–3 Hz/s (mechanical limit), which corresponds to a threshold in Δ P /Δ t ∼ 3–4 mb/min. Moreover, the mechanical tuners, when moving, tend to excite mechanical vibrations in the large drum-like end-plates. While the latter are typically well compensated for by the VCX fast tuners, a relevant optimization work on the refrigeration plant proved to be necessary, in order to reduce the fluctuations of P He , which were initially as high as 30 mb/min, below the mentioned threshold (3–4 mbar/min). 3.1 Limiting He-pressure fluctuations in the refrigerator Both the overall pressure excursions and the rate of pressure change in the SRFQ cryostat were significantly reduced in September–October 2004. This objective was reached: • through a careful setup of the parameters controlling the opening of the cryostat valves, which had to be operated in a continuous filling mode, • keeping the liquid He level constant, by compensating the rf thermal load with a heater in the dewar or, conversely, by increasing the liquid helium production rate. Two compensation setups were implemented, one for the SRFQ cryostat operating alone and one for the three PIAVE cryostats operating together. Figs. 3 and 4 show the result of this optimization process. In Fig. 3 , the variation of the liquid He pressure in the SRFQ cryostat, in a typical working day in June 2004, is shown. Fig. 4 reports an equivalent graph, obtained one year later after the optimization of the refrigerator parameters. As can be seen, both the overall pressure excursions and the maximum values of Δ P /Δ t were significantly decreased. The latter went from more than 30 mb/min to below 2 mb/min, i.e. below the threshold of effective performance of the mechanical tuners. 3.2 Stability performance In the refrigerator conditions shown in Fig. 4 , it was much easier to maintain phase and amplitude lock of each resonator to the master oscillator than was reported earlier [10] for conditions similar to those of Fig. 3 . Figs. 5 and 6 show the phase and amplitude errors of SRFQ1 and SRFQ2, respectively. While in SRFQ2, the VCX fast tuner of which offers a resonant frequency control range of 200 Hz, locking is stable, on SRFQ1, the VCX range of which is 80 Hz, a few jumps of the phase error over a 5 h recording time can still be noted. In nearly all cases, a phase error jump in SRFQ1 coincides with the movement of a slow tuning end-plate, which is moved inward or outward in small steps, depending on the sign of the phase correction required. As mentioned above, it is believed that the stepwise motion induces vibrations on the drum-like end-plate itself, resulting in a short unlock of the cavity phase. This mechanical vibration is damped in a few hundreds ms, as could be observed by a digital oscilloscope. The frequency window of the SRFQ1 VCX must be increased and we plan to do this in the first planned injector stop. However, a few unlocking events can hardly be noted in most nuclear physics experiments; for those few experiments in which this might be a problem, one can plan to inhibit data acquisition during an unlock event. 4 Setup of the relative phase between SRFQ1 and SRFQ2 The relative phase between the two SRFQs can only be set, by looking at beam output energy and beam transmission. We accelerated a beam of 16 O 3+ (PIAVE pilot beam), coming from the Alice ECRIS on the 350 kV platform. Once a proper phase and amplitude stability has been achieved, the two SRFQs can be prepared for beam acceleration. A split SRFQ (two resonators) with additional external bunching requires proper phasing of these three elements. We proceeded in the following way: • Field amplitudes of SRFQs and 3-harmonic buncher were set according to the computed values. • The external buncher was kept off and the relative phase between SRFQ1 and SRFQ2 was found, looking at expected energy gain and beam transmission. • When the proper value of phase between SRFQ1 and SRFQ2 was found, the buncher was switched on and its phase scanned, looking for maximum transmission. The beam output energy was measured through elastic scattering of accelerated ions from a thin Au foil into a Si detector, located at a 25° angle from the beam axis. Fig. 7 shows the expected beam output energy and transmission vs. the phase difference between the two resonators. The transmission is shown in the same graph. In Fig. 8 , the beam energy curve of Fig. 7 (red line in Fig. 8 ) is compared with the experimental values, which reproduce the theoretical curve rather well. The optimum phase difference is marked by the dashed line in both graphs. Optimization of field and phase of the 3-harmonic buncher increases then the overall transmission from 30% to 68% (70% being the computed value). 5 Alignment tolerances The computed SRFQs alignment specifications are better than 0.2 mm on all Cartesian axes, so as not to spoil beam transmission: this holds true for both the alignment between the two SRFQs and between them and the injection line. Once the beam was correctly accelerated, we decided to purposely and stepwise misalign the quadrupole doublet in front of the SRFQs between +1.2 and −1.2 mm and look at beam transmission, so as to check the computed alignment tolerances experimentally. Fig. 9 shows the result of the purposely made misalignment on the vertical axis: it can be seen that, as long as the misalignment is smaller than ±0.2 mm, beam transmission is very marginally affected by that (a few percent only). Acknowledgements We gratefully acknowledge the skilful contribution of the technicians of INFN-LNL, in particular M. De Lazzari and F. Carletto (vacuum system), O. Carletto (VCX fast tuners), E. Bissiato and S. Marigo (mechanics). References [1] A. Pisent, in: Proc. of the Eight Int Conf on Heavy Ion Accelerator Technology, Argonne (IL, USA), October 1998, p. 214. [2] A. Facco, F. Scarpa, V. Zviagintsev, in: Proc. of the Eight Int Conf on Heavy Ion Accelerator Technology, Argonne (IL, USA), October 1998, p. 185. [3] G. Bisoffi et al., in: Proc. of the Eight Int Conf on Heavy Ion Accelerator Technology, Argonne (IL, USA), October 1998, p. 173. [4] G. Bisoffi et al., in: Proc. of EPAC 2000, Vienna, June 2000, p. 324. [5] G. Bisoffi et al., in: Proc. of EPAC 2002, Paris, June 2002, p. 266. [6] G. Bisoffi et al., in: Proc. of the 11th Workshop on Rf-Superconductivity, Lübeck, Travemünde (Germany), September 2003. [7] A. Lombardi et al., in: Proc. of the 1999 Particle Accelerator Conference, New York (USA), May 1999, p. 1324. [8] J.R. Delayen G.J. Dick J.E. Mercereau IEEE Trans. Nucl. Sci. NS-24 3 1977 1759 [9] V. Andreev et al., in: Proc. of EPAC 2000, Vienna, June 2000, p. 2013. [10] G. Bisoffi et al., in: Proc. of LINAC 2004, Lübeck (Germany), August 2004, p. 623.
MoreTranslated text
Key words
RFQ,heavy ion linac,commissioning,superconducting RF
AI Read Science
Must-Reading Tree
Example
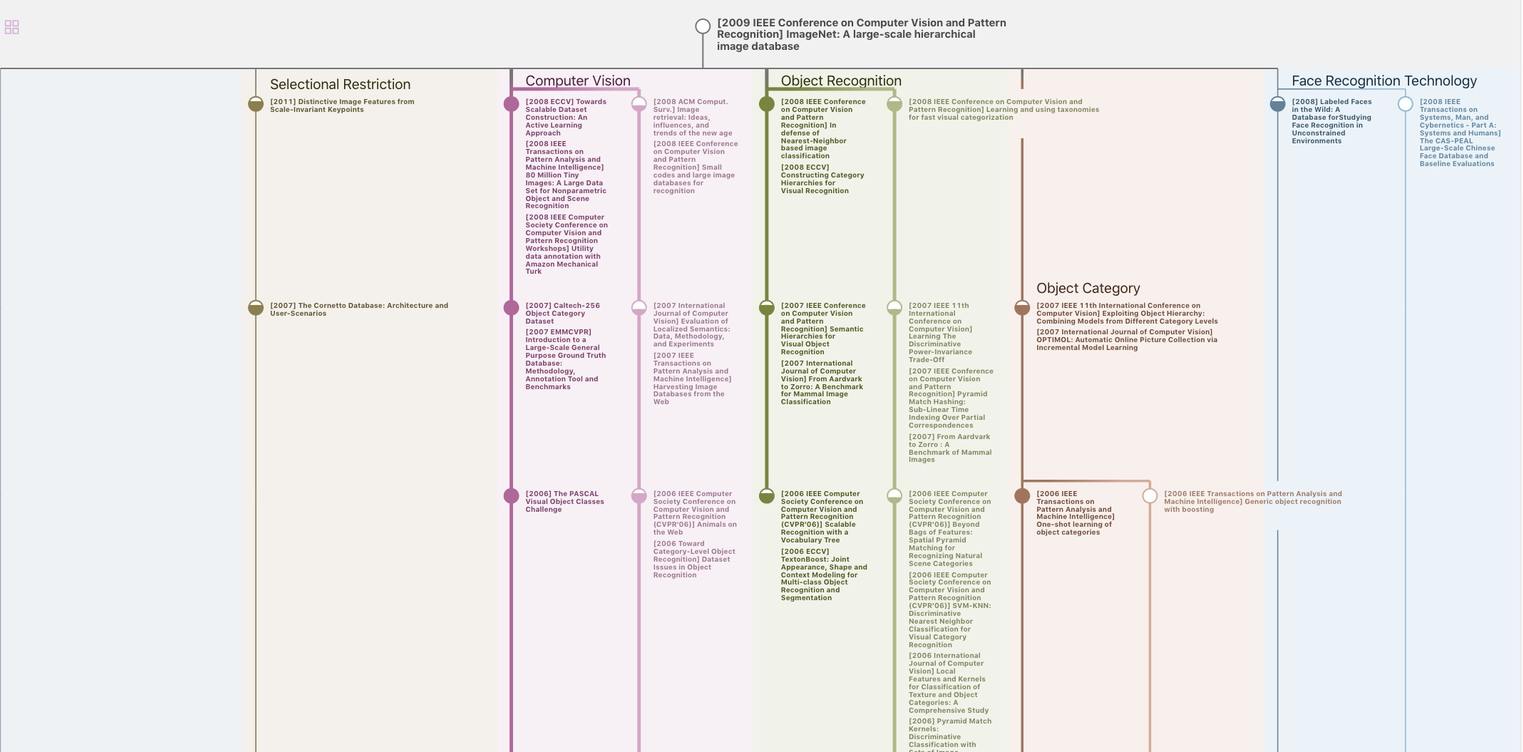
Generate MRT to find the research sequence of this paper
Chat Paper
Summary is being generated by the instructions you defined